Human milk is the primary source of nutrients for newborns, term, and preterm infants. From nutritional, immunological and food safety points of view, human milk is the gold standard as food for children in the first phase of their lives (Jensen, Reference Jensen1999, Reference Jensen2018). Human milk maintains healthy growth and development, improves the gastrointestinal process, favors the mother−child bond (Silva et al., Reference Silva, Cotting, Poltronieri, Balcão, Almeida, Goncalves, Grimaldi and Gioielli2009), and further facilitates the emotional, cognitive and nervous system development of the infant (Calhoun et al., Reference Calhoun, Lunoe, Du and Christensen2000; Bezkorovainy, Reference Bezkorovainy2001). Human milk is rich in biologically active components, such as oligosaccharides, hormones and growth factors that provide the short- and long-term benefits of breastfeeding (Lönnerdal, Reference Lönnerdal2014). These bioactive molecules can affect the microbiota composition, modulate gastrointestinal physiology, enhance intestinal barrier function and stimulate proper development of the immune system (Gila-Diaz et al., Reference Gila-Diaz, Arribas, Algara, Martín-Cabrejas, López de Pablo, Sáenz de Pipaón and Ramiro-Cortijo2019). Moreover, the composition and chemical structure of lipids play a vital role in the superior performance of human milk compared to infant formula.
Despite significant efforts to promote breastfeeding for infants, urbanization and the overall lack of time have reduced the breastfeeding period. Worldwide, only 35% of infants in the newborn to 6-month-old age group are breastfed (Zhao et al., Reference Zhao, Zhao, Du, Binns and Lee2017). The rates of breastfeeding in the US diverge widely along the lines of race, socioeconomic status and ethnicity. According to the National Immunization Survey from the CDC (2017), among children born in 2014, breastfeeding at 6 months after birth, as endorsed by the American Academy of Pediatrics, differed markedly between ethnic groups (Hardison-Moody et al., Reference Hardison-Moody, MacNell, Sa and Bowen2018 and Fig. 1).
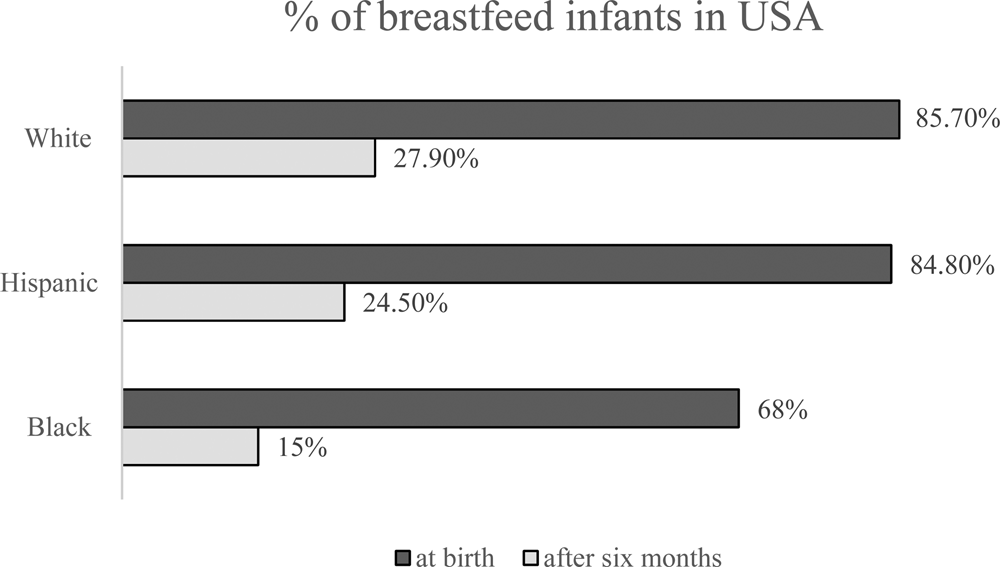
Fig. 1. The percentage of breast feed infants in USA (CDC, 2017).
Notwithstanding the strategies to increase breastfeeding, there will always be a demand for the development of formulas for infants who cannot be breastfed due to medical, social or other reasons. Thus, researchers in this field have attempted to produce an infant formula that can mimic the natural beneficial characteristics of human milk as closely as possible. Whilst all of the nutritional components in human milk have value, the particular importance of the lipid fraction in child development has been highlighted in the literature. The development of a new lipid system with properties analogous to the human milk fat globule is an important step toward reducing the gap between formula-fed and breast-fed babies with regard to neurodevelopment, infectious diseases and cholesterol metabolism. At this time, there is still no large-scale manufacturing process that has been adopted by the dairy industry to process MFGM. Thus, it is important to discuss the particularities of each component of the milk fat globule in order to understand its physical, chemical and nutritional characteristics.
Lipids in infant nutrition
Human milk provides more energy in the form of lactose than does milk of dairy species, nevertheless, milk fat remains the major energy source (40–60%) for breastfed infants in the first stage of life (Koletzko et al., Reference Koletzko, Agostoni, Bergmann, Ritzenthaler and Shamir2011; Demmelmair and Koletzko, Reference Demmelmair and Koletzko2018). Human milk contains 3−5% lipids, of which 98% are triacylglycerol with 88% of fatty acids (Jensen, Reference Jensen1999). Human milk fat is a vital source of essential nutrients for infants, such as the polyunsaturated fatty acids (PUFAs) and long-chain polyunsaturated fatty acids (LC-PUFA) of the n-6 and n-3 series (Delplanque et al., Reference Delplanque, Gibson, Koletzko, Lapillonne and Strandvik2015). It is also involved in the transport of vitamins (A, D, E, and K) and liposoluble hormones (Lönnerdal, Reference Lönnerdal1996). The long-chain polyunsaturated fatty acids (LC-PUFA) such as docosahexaenoic (DHA) and arachidonic acids (ARA) shown in Fig. 2 are fundamental to developing visual acuity in infants and help to improve cognitive function (Jensen, Reference Jensen1999). Although human milk contains at least 170 different triacylglycerol structures, only 30 of them represent 70% of the total human milk fat. Importantly, the lipids are present as fat globules mainly constituted of triacylglycerols surrounded by a structural membrane composed of phospholipids, cholesterol, proteins, and glycoproteins termed as milk fat globule membrane.
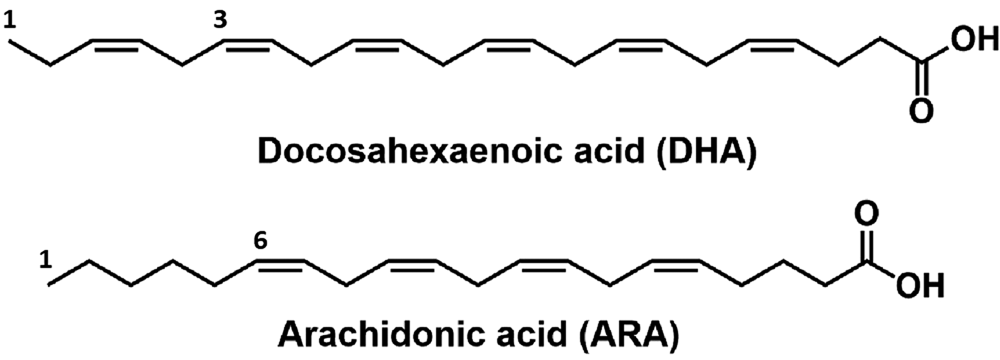
Fig. 2. Structural formulas of Docosahexaenoic acid (DHA) and Arachidonic acid (ARA) fatty acids.
Fatty acid composition
The fatty acid composition of human milk is variable and is influenced by a variety of factors, such as diet, duration of pregnancy, maternal parity, and stage of lactation. The most important variable to determine fatty acid composition is the maternal diet. Table 1 presents the fatty acid composition of human milk from American mothers; these results were obtained from Yuhas et al. (Reference Yuhas, Pramuk and Lien2006). The main fatty acids are oleic acid (32.8%), palmitic acid (19.3%), linoleic acid (14.8%), myristic acid (4.9%), and stearic acid (6.2%).
Table 1. Fatty acid composition of human milk in the United States
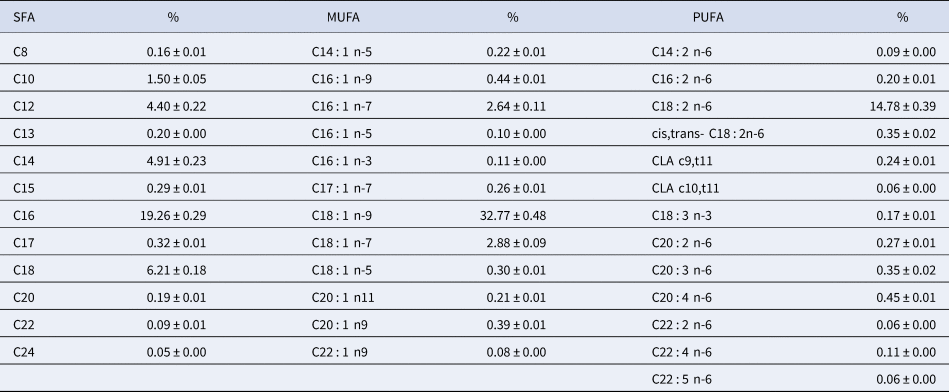
SFA, saturated fatty acid; MUFA, monounsaturated fatty acids; PUFA, polyunsaturated fatty acids.
Adapted from Yuhas et al. (Reference Yuhas, Pramuk and Lien2006).
The saturated fatty acids present in infant diets mostly have 12, 14, 16, and 18 carbon chain lengths (Delplanque et al., Reference Delplanque, Gibson, Koletzko, Lapillonne and Strandvik2015). Besides the nutritional role, the saturated fatty acids may have physiological relevance, such as the occurrence of palmitic acid in pulmonary surfactant (schmidt et al., 2002). In studies in vitro or with animals, capric acid often exhibits bactericidal effects (Bergsson et al., Reference Bergsson, Arnfinnsson, Steingrímsson and Thormar2001) whilst arachidic and behenic acids have immunomodulatory effects (Tollin et al., Reference Tollin, Bergsson, Kai-Larsen, Lengqvist, Sjövall, Griffiths, Skúladóttir, Haraldsson, Jörnvall, Gudmundsson and Agerberth2005), and protein acylation occurs for myristic or palmitic acids (Rioux et al., Reference Rioux, Pédrono and Legrand2011). These saturated fatty acid properties should be worthy of further exploration in humans and infants.
The medium-chain fatty acids (8–10 carbons) also have importance for infant health. Caprylic and capric acids (C8 and C10) are used to facilitate fat absorption in preterm babies through the intestine due to intestinal immaturity; however, there is no evidence demonstrating benefit for energy balance or growth (Lapillonne et al., Reference Lapillonne, Groh-Wargo, Gonzalez and Uauy2013).
Monounsaturated fatty acids (MUFA) are the prevalent group of fatty acids in breast milk and infant formula. The primary monounsaturated fatty acids are oleic acid (C18 : 1n-9) and palmitoleic acid (C16 : 1n-7) (Table 1). Besides the usual functions of fatty acids (source of energy and structural components), oleic acid performs a vital role in the reduction of the melting point of triacylglycerides, thus providing the liquidity required for the formation, transport, and metabolism of milk fat globules (Jensen, Reference Jensen1999).
Polyunsaturated fatty acids (PUFA) such as linoleic acid (LA) (18 : 2n-6) and α-linolenic acid (ALA) (18 : 3n-3) are precursors of long-chain polyunsaturated fatty acids (LCPUFA) such as arachidonic acid (AA; 20 : 4n- 6) and docosahexaenoic acid (DHA; 22 : 6n-3). These fatty acids are necessary for the growth of the brain and the retina during perinatal development and influence metabolic processes, such as decreasing plasma cholesterol (Jensen, Reference Jensen1999). Human milk contents of LA and ALA vary according to the maternal intakes of these fatty acids. The European Food Safety Authority (EFSA) recommends amounts of LA and ALA for infant formula are, respectively, 4.5 and 0.5% of energy content, with high levels set at respectively 10.8 and 0.9% of energy (EFSA, 2014). Due to the limited capacity of term infants to synthesize LCPUFA, such as AA, DHA, and EPA, they depend on the dietary supply during the first months of life (Sahin-Yesilcubuk and Akoh, Reference Sahin-Yesilcubuk and Akoh2017). Supplementation of infant formulas with long-chain LCPUFAs (AA and DHA) at levels resembling human milk is recommended by the Food and Agriculture Organization/World Health Organization since LCPUFAs provide biochemical and functional benefits to the neonate. DHA and ARA-rich oils from single-cell organisms are also potential LCPUFA sources to supplement infant formula (Alvares and Akoh, Reference Alvares and Akoh2016). Single-cell oils are nutraceutical ingredients of non-animal origin produced by oleaginous microorganisms such as algae, fungi, and bacteria currently used in infant formulas to supplement LCPUFAs (Ratledge, Reference Ratledge2004; Papanikolaou and Aggelis, Reference Papanikolaou and Aggelis2011; Ochsenreither et al., Reference Ochsenreither, Glück, Stressler, Fischer and Syldatk2016).
Triacylglycerol composition
The triacylglycerols (TAG) in human milk fat have a distinct molecular structure (Mazzocchi et al., Reference Mazzocchi, D'Oria, De Cosmi, Bettocchi, Milani, Silano and Agostoni2018). In human milk fat, the sn-2 position of the TAG is predominantly occupied by saturated fatty acids (SFA), while the majority of unsaturated fatty acids (UFA) are distributed at the sn-1,3 positions (Fig. 3). Palmitic acid (16 : 0) is the primary saturated fatty acid (20–25%), and around 60–86% of 16 : 0 is esterified at the sn-2 position of triacylglycerols (β-position) (Innis et al., Reference Innis, Nelson, Lwanga, Rioux and Waslen1996). Several studies over the last three decades have shown that the 16 : 0 distribution at the central position (sn-2) in human milk fat promotes better absorption of lipids as well as calcium in term newborns and preterm infants (Carnielli et al., Reference Carnielli, Luijendijk, van Beek, Boerma, Degenhart and Sauer1995; Delplanque et al., Reference Delplanque, Gibson, Koletzko, Lapillonne and Strandvik2015). During digestion in newborns and infants, the pancreatic and gastric lipases selectively hydrolyze fatty acids at the sn-1,3 positions, producing free fatty acids and a 2-monoacylglycerol. The monoacylglycerol containing palmitic acid (2-palmitoyl glycerol) is more efficiently absorbed than palmitic acid in its free form. This TAG structure is a crucial factor in mineral and fat absorption in the first period of an infant's life and beyond. The palmitic acid in free form has low absorption in the intestinal lumen, whereas short-chain free fatty acids, and 18-carbon free fatty acids (such as oleic and linoleic acid) are well-absorbed (Carnielli et al., Reference Carnielli, Luijendijk, Van Goudoever, Sulkers, Boerlage, Degenhart and Sauer1996; Kennedy et al., Reference Kennedy, Fewtrell, Morley, Abbott, Quinlan, Wells, Bindels and Lucas1999). This is due to the melting point of palmitic acid (61–65°C) being higher than body temperature (Gunstone, Reference Gunstone and Shahidi2009), as well as the strong tendency to form insoluble soaps with divalent cations such as calcium and magnesium at the alkaline pH of the intestine (de Fouw et al., Reference de Fouw, Kivits, Quinlan and van Nielen1994). By contrast, vegetable oils and some mammalian milk fats present SFAs that are primarily located at the sn-1,3 positions of TAG (Mu and Høy, Reference Mu and Høy2004) and unsaturated fatty acids are esterified at sn-2 (Xu, Reference Xu2000) (Fig. 1), which is different from the location in human milk fat, causing a loss of minerals and energy in the infant (Carnielli et al., Reference Carnielli, Luijendijk, Van Goudoever, Sulkers, Boerlage, Degenhart and Sauer1996; Kennedy et al., Reference Kennedy, Fewtrell, Morley, Abbott, Quinlan, Wells, Bindels and Lucas1999; López-López et al., Reference López-López, Castellote-Bargalló, Campoy-Folgoso, Rivero-Urgël, Tormo-Carnicé, Infante-Pina and López-Sabater2001).
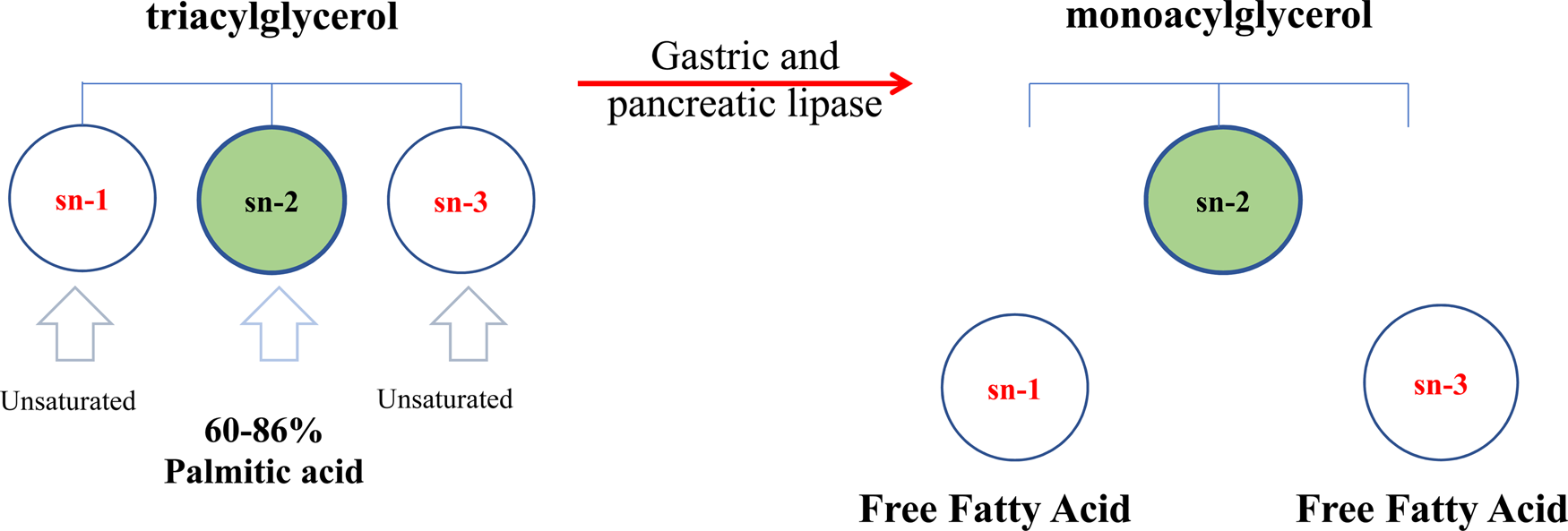
Fig. 3. Schematic triacylglycerol structure of human milk fat. (Adapted from Mehrotra et al., Reference Mehrotra, Sehgal and Bangale2018).
Lipid modification technology, particularly interesterification, is used to design lipids with specific TAG structure. Previous research in neonatal nutrition has suggested that developing more biomimetic infant formulas with a TAG structure more similar to that of human milk would lead to positive outcomes in terms of metabolism programming (Oosting et al., Reference Oosting, van Vlies, Kegler, Schipper, Abrahamse-Berkeveld, Ringler, Verkade and van der Beek2014). Many researchers have shown that the lipid structure of human milk can be artificially produced by enzymatic interesterification using as the catalyst the specific lipase sn-1,3 (Quinlan and Chandler, Reference Quinlan and Chandler1992; Mukherjee and Kiewitt, Reference Mukherjee and Kiewitt1998; Yang et al., Reference Yang, Xu, He and Li2003). Several types of oils and fats are used as a substrate for the production of structured lipid substitute for human milk fat, such as tripalmitin, lard fat, bovine milk fat, soybean oil, canola oil, borage oil, sunflower oil, safflower oil. Table 2 presents the main research in lipid modification to produce a structured lipid substitute for human milk fat in the past five years.
Table 2. An updated summary of enzyme-catalyzed synthesis of human milk fat structured lipids
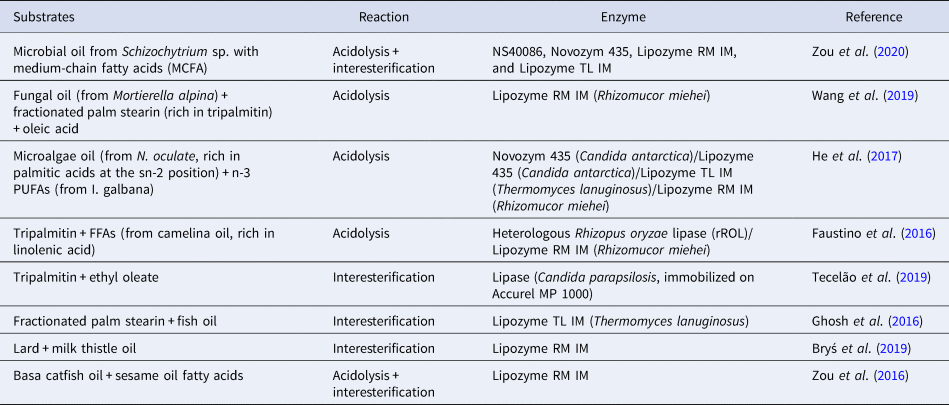
Yaron and collaborators demonstrated an increment in the fecal occurrence of Lactobacillus and Bifidobacterium genera in infants that, for 6 weeks, received a formula supplemented with β-16 : 0 compared to a control group that received formula containing vegetable-sourced 16 : 0 (Yaron et al., Reference Yaron, Shachar, Abramas, Riskin, Bader, Litmanovitz, Bar-Yoseph, Cohen, Levi, Lifshitz, Shamir and Shaoul2013). The mechanisms behind this study need to be better explored but suggest that the position of palmitic acid on the TAG may have an impact on the gut microbiota.
Infant formulas marketed in the US typically include palm, coconut, soybean, and sunflower oils as the primary lipid sources. The development of a structured lipid that is similar to human milk fat using low cost, sustainable oils such as single-cell oils would, therefore, be a significant advance for the infant formula industry and would offer high technology formulas for those infants who do not have access to breastfeeding and must depend on infant formula.
Milk fat globule membrane
The human milk fat globule has aroused the interest of many researchers recently. It is a heterogeneous structure varying in diameter, triglyceride content, membrane, and fatty acid composition. The diameter of these globules generally ranges from 0.2 to 15 μm, with an average diameter between 4 and 5 μm, and milk fat globules in other mammalian species do not show differences in globule diameters ranging between 3 and 5 μm (Lopez and Ménard, Reference Lopez and Ménard2011). The lipid droplets (TAG-rich core) of milk fat globules (including those found in breast milk) are stabilized by a 3-layered membrane called a milk fat globule membrane (MFGM), which maintains triglyceride dispersion in the aqueous phase of the milk (Fig. 4).
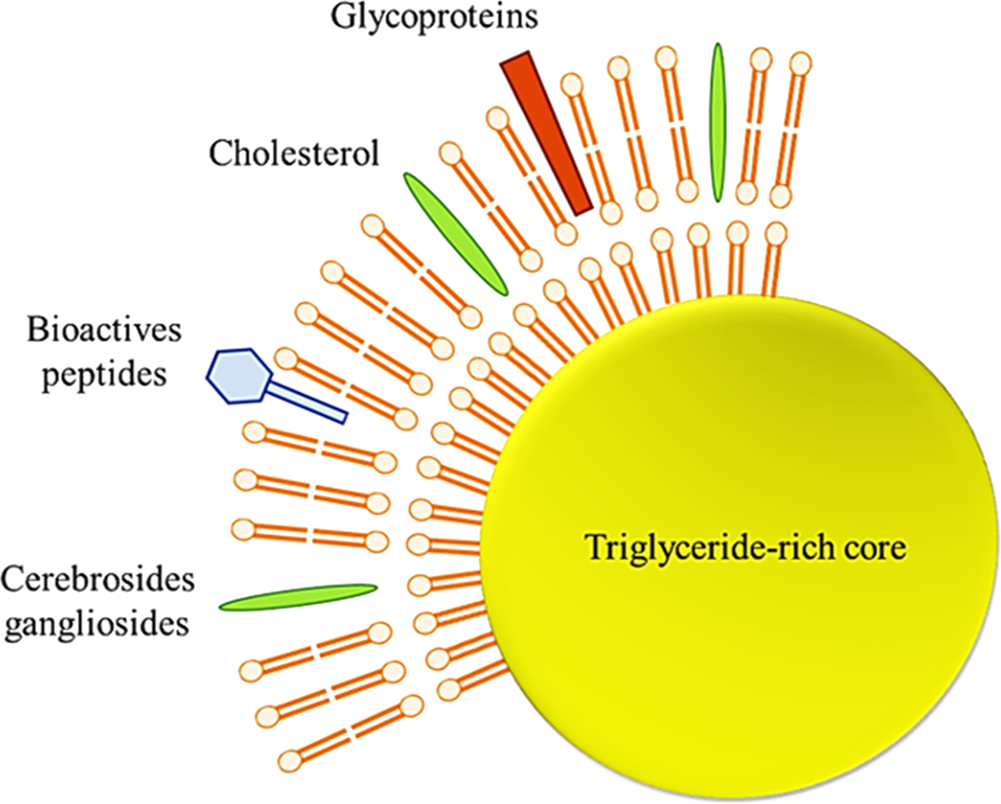
Fig. 4. Schematic structure of milk fat globule (Adapted from Koletzko, Reference Koletzko2016).
Preclinical and clinical studies regarding MFGM and its components in relation to resistance to infections, cognitive development, establishment of gut microbiota and infant metabolism was critically examined in a recent review by Brink and Lönnerdal (Reference Brink and Lönnerdal2020). MFGM is highly structured and contains various components, such as specific proteins (mainly glycoproteins), polar lipids (phospholipids and glycosphingolipids), and apolar lipids (cholesterol and cerebrosides) (Dewettinck et al., Reference Dewettinck, Rombaut, Thienpont, Le, Messens and Van Camp2008). This membrane, about 10–20 nmin cross-section, acts as an emulsifier and protects the globules from coalescence and enzymatic degradation.
Although MFGM proteins account for only 1–4% of total milk proteins and 1% of the total globule mass, more than five hundred proteins with diverse functions have been identified by proteomics analysis (Cao et al., Reference Cao, Kang, Yang, Li, Wu, Han, Meng, Wu and Yue2018). Some have been well described, such as mucins, xanthine oxidoreductase, glycosylated butyrophilin, lactadherin, CD proteins, the non-glycosylated adipophilin, and fatty acid binding proteins (Lee et al., Reference Lee, Padhi, Hasegawa, Larke, Parenti, Wang, Hernell, Lönnerdal and Slupsky2018). Several MFGM proteins, such as lactadherin, butyrophilin, and MUC1, have been shown to have antibacterial and antiviral activity in vitro (Lönnerdal, Reference Lönnerdal2016).
Previous studies have supported the concept of the antiviral activity of MFGM proteins. Lactadherin concentration in human milk was associated with activity against rotavirus (Newburg et al., Reference Newburg, Peterson, Ruiz-Palacios, Matson, Morrow, Shults, Guerrero, Chaturvedi, Newburg, Scallan, Taylor, Ceriani and Pickering1998). Kvistgaard et al. (Reference Kvistgaard, Pallesen, Arias, López, Petersen, Heegaard and Rasmussen2004) demonstrated the inhibitory effects of lactadherin and mucin extracted from bovine MFGM in rotavirus infection. MFGM proteins also reduce the incidence of diarrhea in infants that received bovine (MFGM)-enriched protein fraction as a complementary food (Zavaleta et al., Reference Zavaleta, Kvistgaard, Graverholt, Respicio, Guija, Valencia and Lönnerdal2011). Timby et al. (Reference Timby, Domellof, Hernell, Lonnerdal and Domellof2014) added bovine MFGM fraction to infant formula, fed infants with this formula for six months of age and then followed up to 1 year of age. A significant reduction in illness (acute otitis media) and prescriptions of antipyretics in the infants fed the MFGM-supplemented formula was found compared with infants fed regular formula, with no difference compared to breastfed infants. These authors concluded that the addition of MFGM to infant formula had significant health benefits for infants. These observations endorse the role of human milk MFGM proteins in the defense against infection in breastfed infants.
MFGM proteins are influenced by several maternal factors, such as the period of lactation, environmental conditions, and the different species. Not surprisingly, analysis of human colostrum revealed levels of proteins with potential antimicrobial function considerably higher than in mature human milk, which may compensate for immature neonatal immunity (Cao et al., Reference Cao, Kang, Yang, Li, Wu, Han, Meng, Wu and Yue2018).
The polar lipids of the MFGM include phospho- and sphingolipids, that are amphiphilic molecules with a hydrophobic tail and a hydrophilic head group. Phospholipids are complex mixtures of more than 30 molecular species of phosphatidylcholine, phosphatidylethanolamine, phosphatidylserine, phosphatidylinositol, and sphingophospholipids. Phospholipid molecules consist of a glycerol backbone on which two fatty acids are esterified, and a phosphate residue with different organic groups (choline, serine, ethanolamine, among others) may be linked on the third hydroxyl group. Thus, phosphatidylethanolamine, phosphatidylcholine, and sphingomyelin are the major milk phospholipids, representing about 90% of all MFGM polar lipids.
Phosphatidylcholine represents up to 35% of the total of phospholipids, and its primary function is to maintain the permeability of the membrane (Dewettinck et al., Reference Dewettinck, Rombaut, Thienpont, Le, Messens and Van Camp2008; Jiménez-Flores et al., Reference Jiménez-Flores, Higuera-Ciapara and Pouliot2009) due to its flexible morphology. Phosphatidylethanolamine constitutes up to 30% of the total phospholipids in the MFGM, and its mostly bound to unsaturated fatty acids (68%) with high levels of oleic acid (C18 : 1) and linoleic acid (C18 : 2) (Sánchez-Juanes et al., Reference Sánchez-Juanes, Alonso, Zancada and Hueso2009).
Sphingomyelin is an important constituent of the milk phospholipid fraction, due to its high concentration in the MFGM (up to 35% of phospholipids) and its implication in many beneficial effects on human health, such as neuronal development in infants and protection of neonates from bacterial infections (Bourlieu et al., Reference Bourlieu, Bouzerzour, Ferret-Bernard, Bourgot, Chever, Ménard, Deglaire, Cuinet, Ruyet, Bonhomme, Dupont and Huërou-Luron2015). Interestingly, about 97% of all MFGM sphingomyelins are composed of medium-chain or long-chain saturated fatty acids (C16 : 0, C22 : 0, C23 : 0, and C24 : 0) with high melting temperatures. More rarely, 17% or more of milk sphingomyelins contain C23 : 0 fatty acids (Dewettinck et al., Reference Dewettinck, Rombaut, Thienpont, Le, Messens and Van Camp2008). This unique characteristic of milk sphingomyelins suggests a specific, probably biological, function well beyond its simple structural role in the MFGM. Feeding rats pups with bovine milk sphingomyelins has been shown to improve markers of gut maturation (Motouri et al., Reference Motouri, Matsuyama, Yamamura, Tanaka, Aoe, Iwanaga and Kawakami2003), providing evidence of the importance of dietary sphingomyelin for newborn gut development. Studies have shown a heterogeneous distribution of polar lipids in the outer bilayer of the human MFGM, with the lateral segregation of sphingomyelin in domains that could have functional roles (Lopez and Ménard, Reference Lopez and Ménard2011; Zou et al., Reference Zou, Guo, Huang, Jin, Cheong, Wang and Xu2012).
MFGM fragments are considered functional ingredients as natural emulsifiers (Schmelz, Reference Schmelz2000) due to the amphiphilic nature of phospholipids, their effectiveness at low concentrations and their original function in stabilizing the fat globules in whole milk (Corredig and Dalgleish, Reference Corredig and Dalgleish1998). Kanno (Reference Kanno1989) investigated the emulsifying properties (foam and emulsion stability, emulsion capability, and whip ability) of MFGM isolates by reconstituting the milk fat globules and concluded that the amounts of MFGM material (20–80 mg g/1fat) significantly affected the properties of the reconstituted emulsions.
MFGM also provides considerable amounts of cholesterol. Cholesterol is a crucial building block for all cell membranes. It affects the development of myelin in the central and peripheral nervous systems, assists as the substratum for the synthesis of bile acids, lipoproteins, vitamin D, hormones, and oxysterols that modulate cholesterol, lipid, and glucose homeostasis (Koletzko, Reference Koletzko2016). The consumption of cholesterol by breastfeeding is associated with higher plasma concentrations of total and low-density lipoprotein cholesterol (LDL-cholesterol) compared to formula-fed infants (Hernell et al., Reference Hernell, Fewtrell, Georgieff, Krebs and Lönnerdal2015). However, this difference in serum cholesterol disappears in childhood (Fomon et al., Reference Fomon, Rogers, Ziegler, Nelson and Thomas1984) and adolescence and, after that, previously breastfed infants have slightly lower serum cholesterol than their formula-fed counterparts (Owen et al., Reference Owen, Whincup, Odoki, Gilg and Cook2002).
Although MFGM is only some nanometers thick, and the diameters of the milk fat globules are in the micrometer range, MFGM seems to be essential for the physiological properties of human milk. MFGM might contribute to the differences in outcome between breastfed and formula-fed infants, which continue to exist even though the difference has become smaller with improved formula qualities (Koletzko and Shamir, Reference Koletzko and Shamir2016).
Human vs. bovine MFGM
MFGM is a component of bovine milk that historically has been discarded with the milk fat during the production of infant formula. Commercially available MFGM isolates are predominantly bovine sourced, and the polar lipids and proteins should be better explored as an emulsifier or stabilizer, combining technological and nutritional functionality (Dewettinck et al., Reference Dewettinck, Rombaut, Thienpont, Le, Messens and Van Camp2008). Human and bovine MFGM have similar polar lipid assemblies, with hundreds of different molecules of polar lipids, varying the polar heads and acyl chains. However, they differ on two major points: human MFGM contains, on average, more sphingomyelin than bovine, and human MFGM acyl chains are, on average, more unsaturated than bovine (Lopez and Ménard, Reference Lopez and Ménard2011). Human MFGM is rich in LC-PUFA of the omega 3 series, such as docosahexaenoic acid (DHA), which is considered as essential for cerebral and visual development during the neonatal period (Yehuda et al., Reference Yehuda, Rabinovitz and Mostofsky2005). As stated above, the capacity of bioconversion of linolenic acid and alpha-linolenic acid into long-chain polyunsaturated fatty acids is limited during the neonatal period, inducing dependence on the dietary intakes from human milk lipids (Lonnerdal, 2016).
There has been little study of non-cattle MFGM, so it is of note that Ji et al. (Reference Ji, Xu, Cui, Ma and Zhou2019) recently compared the MFGM of five different species (yak, camel, bovine, buffalo, and goat). These kinds of milk differ in the MFGM content as follows: yak milk presented the highest amount (0.23%) followed by bovine milk (0.18%), buffalo milk (0.15%), camel milk (0.13%) and goat milk with the lowest amount of MFGM (0.068%). The protein content of yak milk was the highest (425.1 mg/g) followed by buffalo MFGM (416.5 mg/g), bovine MFGM (378.7 mg/g), camel MFGM (348.1 mg/g) and goat MFGM (302.6 mg/g) (Ji et al., Reference Ji, Xu, Cui, Ma and Zhou2019). Lamothe et al. (Reference Lamothe, Robitaille, St-Gelais and Britten2008) studied the effects of washing treatment on phospholipids and milk fat globule membrane but did not find differences in the sphyngomielin contents of bovine and caprine milk.
Advances in proteomics technology, especially quantitative proteomics, have allowed an increased knowledge and characterization of MFGM proteins (Sui et al., Reference Sui, Zhao, Wang, Zhang, Guo, Yu and Li2014). Thus, proteomics has been successfully applied to studies of human, bovine, and goat MFGM proteins (Bianchi et al., Reference Bianchi, Puglia, Landi, Matteoni, Perini, Armini, Verani, Trombetta, Soldani, Roncada, Greppi, Pallini and Bini2009; Spertino et al., Reference Spertino, Cipriani, De Angelis, Giuffrida, Marsano and Cavaletto2012). Most of the published studies on MFGM proteins have been dedicated to the bovine species. Major MFGM bovine proteins include mucin 1, xanthine oxidase, CD36, butyrophilin, adipophilin, PAS6/7 (lactadherin), and fatty acid-binding protein (Cavalleto et al., Reference Cavalleto, Giuffrida, Conti and Bösze2008). Ye et al. (Reference Ye, Singh, Taylor and Anema2002) characterized bovine MFGM using one- and two-dimensional SDS-PAGE to determine the effect of heat treatment on MFGM proteins from early, mid-, and late-season and they found that xanthine oxidase and butyrophilin formed aggregates via intermolecular disulfide bonds after the heating process. The aggregation reduces the physical stability of the protein in question, not only leading to a loss of activity but also other critical problems such as toxicity and immunogenicity (Wang et al., Reference Wang, Singh, Li, Toler, King and Nema2012).
In addition to the typical MFGM proteins such as xanthine oxidase, butyrophilin and fatty acid-binding protein, other proteins have been mapped in human MFGM. These include the following: the IgM m chain, the IgA a chain, the HLA class I heavy chain, immunoglobulin light chains, secretory piece, J chain, actin, a acid glycoprotein, albumin and casein, with particular attention given to the pattern of apolipoproteins E, A-I, A-II, and H (Reinhardt and Lippolis, Reference Reinhardt and Lippolis2006; Cavalleto et al., Reference Cavalleto, Giuffrida, Conti and Bösze2008). Higher concentrations of four proteins involved in the mucosal defense system (IgA, CD14, LTF, and LYZ) were found more in human milk than in bovine milk. These differences in concentration between species may be related to differences in the development of the immune system of babies and calves (Cavalleto et al., Reference Cavalleto, Giuffrida, Conti and Bösze2008).
MFGM in infant formulas
Infant formula development has undergone many modifications in previous decades, with the goal of improving nutritional performance in order to be more similar to human milk. These changes, some of which are relatively recent, include modifications of whey and casein as well as addition of taurine, MFGM, nucleotides, docosahexaenoic acid, arachidonic acid, prebiotics (fructooligosaccharides/galactooligosaccharides) and lutein (Koletzko, 2016). Despite these improvements, differences remain between breastfed infants and formula-fed infants with regard to short-term (e.g., illnesses, cognitive development) and long-term (e.g., obesity, diabetes, cardiovascular disease) outcomes.
When nonfat milk is used as the base for infant formula, and animal fat is replaced with vegetable oils, the levels of MFGM are usually very low (Brink and Lönnerdal, Reference Brink and Lönnerdal2020) with a lack of essential bioactive molecules. Such commercial infant formulas contain lipid droplets that have distinct interfacial compositions (such as citrate glycerides, monoglycerides, or diglycerides) compared to human milk. The droplets in infant formulas are typically much smaller than breast milk fat globules, with particles diameter range around 0.3–0.8 μm and a higher surface area (from about 20−40 m2 g1 fat) with an interfacial layer composed primarily of dairy proteins or surfactants (Lopez and Ménard, Reference Lopez and Ménard2011). The smaller lipid droplets found in infant milk formulas can be attributed to homogenization, a stage involved in the production of these processed products (Wei et al., Reference Wei, Jin and Wang2019a, Reference Wei, Yang, Yang, Wang, Yang, Jin, Wang, Lai and Wang2019b).
The infant formula lipid macrostructure, such as lipid droplets and the interfacial composition, has recently been reported to impact on metabolic programming (Oosting et al., Reference Oosting, van Vlies, Kegler, Schipper, Abrahamse-Berkeveld, Ringler, Verkade and van der Beek2014). The addition of MFGM fragments and/or phospholipids from bovine milk at the surface of processed lipid droplets is an efficient conveyor of energy, and also conveys some low-digestible bioactive complex lipids from the MFGM. Over the last decade, several research groups and manufacturers have investigated the addition of ingredients rich in MFGM or complex lipids to infant formula in order to improve growth and development outcomes in infants (Oosting et al., Reference Oosting, Kegler, Wopereis, Teller, van de Heijning, Verkade and van der Beek2012, Reference Oosting, van Vlies, Kegler, Schipper, Abrahamse-Berkeveld, Ringler, Verkade and van der Beek2014; Billeaud et al., Reference Billeaud, Puccio, Saliba, Guillois, Vaysse, Pecquet and Steenhout2014; Timby et al., Reference Timby, Domellof, Hernell, Lonnerdal and Domellof2014, Reference Timby, Hernell, Vaarala, Melin, Lönnerdal and Domellöf2015; Guan et al., Reference Guan, MacGibbon, Fong, Zhang, Liu, Rowan and McJarrow2015; Hernell et al., Reference Hernell, Timby, Domellöf and Lönnerdal2016; Reis et al., Reference Reis, Bermingham, Reis, Deb-Choudhury, MacGibbon, Fong, McJarrow, Bibiloni, Bassett and Roy2016).
Oosting et al. (Reference Oosting, Kegler, Wopereis, Teller, van de Heijning, Verkade and van der Beek2012, Reference Oosting, van Vlies, Kegler, Schipper, Abrahamse-Berkeveld, Ringler, Verkade and van der Beek2014) in animal studies observed that infant formula containing TAG from vegetable origin and covered by polar lipids from the MFGM (a concept infant formula) reduced fat accumulation as well as fasting plasma leptin, resistin, glucose, and lipids (TAG and total cholesterol) in adult animals fed with a western diet, compared to the group fed with standard infant formula. The same group (Baars et al., Reference Baars, Oosting, Engels, Kegler, Kodde, Schipper, Verkade and van der Beek2016) studied the potential role played by large lipid droplets only, of polar lipid-coating only or the combination of both in the protective effect against obesity in later life. They concluded that a diet containing a combination of large lipid droplets with a coating of MFGM mimicking the lipid droplet architecture as present in mammalian milk prevents body fat accumulation in aniumal models when challenged with a moderate western diet during adolescence and adulthood. Although the mechanisms remain unclear, these animal studies showed that early nutrition with MFGM is associated with sustained effects on later life obesity.
The supplementation of infant formula with MFGM has shown several positive results in clinical studies, such as behavioral improvement and regulation in preschool children (Veereman-Wauters et al., Reference Veereman-Wauters, Staelens, Rombaut, Dewettinck, Deboutte, Brummer, Boone and Le Ruyet2012). A randomized clinical trial with infants below 2 months of age presented an improvement in the neurocognitive development when they received MFGM-supplemented, low-energy, low-protein experimental infant formula compared to standard formula. The cognitive score at 12 months of age was significantly higher in the MFGM-supplemented experimental infant formula group than in the standard infant formula group and, furthermore, was not significantly different from that of the breastfed group (Timby et al., Reference Timby, Domellof, Hernell, Lonnerdal and Domellof2014).
There are several pieces of evidence that MGFM components influence brain development, with tremendous differences compared with formula-fed newborns (Gurnida et al., Reference Gurnida, Rowan, Idjradinata, Muchtadi and Sekarwana2012; Oshida et al., Reference Oshida, Shimizu, Takase, Tamura, Shimizu and Yamashiro2003; Tanaka et al., Reference Tanaka, Hosozawa, Kudo, Yoshikawa, Hisata, Shoji, Shinohara and Shimizu2013). Polar lipid supplementation could reduce the gap in neuronal functions such as cognitive performance of infant fed newborns, behavioral development, and myelination-promoting markers between breastfed and formula-fed infants. All these findings even pose the idea that MFGM supplementation has a beneficial effect on neural functions throughout life. These benefits to neurodevelopment may be associated with the ganglioside content of the MFGM, considering the high ganglioside content in nervous tissue, the high requirement in the perinatal period due to rapid brain growth and the demonstrated uptake of dietary gangliosides (Palmano et al., Reference Palmano, Rowan, Guillermo, Guan and McJarrow2015). In a clinical trial study Gurnida et al. (Reference Gurnida, Rowan, Idjradinata, Muchtadi and Sekarwana2012) compared infants fed with an experimental formula enriched with gangliosides to a group fed a standard formula. The authors observed an improvement in hand and eye coordination in those infants receiving the formula containing the gangliosides, and this improvement was correlated with an increase in serum ganglioside levels. In a recent large study, multiple neurodevelopmental tests were conducted in order to investigat the performance of infants receiving infant formula enriched with MFGM-10 and lactoferrin (Li et al., Reference Li, Zhang, Fu, Wang and Zhang2019). It was observed that infants receiving formula with added bovine MFGM and bovine lactoferrin had an accelerated neurodevelopmental profile at day 365 and improved language subcategories at day 545.
Another recent study revealed a significant difference in the fat globule size and membrane structure between the human milk and infant formulas supplied with the MFGM supplemented products (Bourlieu et al., Reference Bourlieu, Deglaire, De Oliveira, Ménard, Le Gouar, Carrière and Dupont2017). The size and structure of fat globule in the infant formula supplemented with MFGM is interesting yet rarely reported (Wei et al., Reference Wei, Jin and Wang2019a, Reference Wei, Yang, Yang, Wang, Yang, Jin, Wang, Lai and Wang2019b). Given the aforementioned protective effects of MFGM, new studies have shown that the supplementation of MFGM formula might prove beneficial within the developing intestine. The supplementation of infant formulas with MFGM also resulted in a bactericidal effect against various foodborne diseases (Bhinder et al., Reference Bhinder, Allaire, Garcia, Lau, Chan, Ryz, Bosman, Graef, Crowley, Celiberto, Berkmann, Dyer, Jacobson, Surette, Innis and Vallance2017). Studies in rats showed enrichment of Gram-negative Bifidobacterium in feces of animals that received supplementation with bovine MFGM (Lee et al., Reference Lee, Padhi, Hasegawa, Larke, Parenti, Wang, Hernell, Lönnerdal and Slupsky2018). Fragments of MFGM that escape digestion in the lumen may proceed to the large intestine and support the colonization of microbial communities (Bourlieu et al., Reference Bourlieu, Bouzerzour, Ferret-Bernard, Bourgot, Chever, Ménard, Deglaire, Cuinet, Ruyet, Bonhomme, Dupont and Huërou-Luron2015). Evaluation of the colonic bacteria of germ-free mice fed two different types of emulsifiers, soy lecithin vs. MFGM phospholipids (Lacprodan® PL-20, Arla, Denmark), revealed that MFGM phospholipids tended to enrich Porphyromonadaceae, while soy lecithin tended to enrich Enterobacteriaceae and Enterococcaceae. In a distinctive study, rat pups fed a formula supplemented with this same bovine MFGM presented an increased gut microbial species richness and uniformity compared with rat pups fed a formula containing vegetable fat.
Bacteria (including Lactobacillus) present in milk have been shown to preferentially associate with the MFGM utilizing glycan adhesion factors that enable bacteria to bind to mucin (Guerin et al., Reference Guerin, Burgain, Gomand, Scher and Gaiani2019). Besides, bacteria with higher surface hydrophobicity, such as L. reuteri, are better able to adhere to the MFGM (Brisson et al., Reference Brisson, Payken, Sharpe and Jiménez-Flores2010), a phenomenon that is related to properties of the bacterial cell surface. In this context, several patents for utilizing the MFGM as a probiotic carrier have been published and recently reviewed with a focus on lactic acid bacteria (Guerin et al., Reference Guerin, Burgain, Gomand, Scher and Gaiani2019).
Infants fed with formula showed deficits in intestinal development compared with breastfed infants, however, this deficiency could be overcome with the addition of MFGM to the infant formula (Bhinder et al., Reference Bhinder, Allaire, Garcia, Lau, Chan, Ryz, Bosman, Graef, Crowley, Celiberto, Berkmann, Dyer, Jacobson, Surette, Innis and Vallance2017). In a recent clinical trial study, He et al. (Reference He, Parenti, Grip, Lönnerdal, Timby, Domellöf, Hernell and Slupsky2019) characterized the fecal microbiome and metabolome of infants fed a bovine MFGM supplemented formula and compared it with the standard formula used with a breastfed reference group. Their results showed an influence of MFGM on the fecal metabolome that may be associated with gut microbial activity and function.
While researchers are still making efforts to comprehend the functionalities of MFGM, manufacturers have already supplemented formulas with fragments/extracts of bovine MFGM and/or phospholipids-enriched materials (Ramiro-Cortijo et al., Reference Ramiro-Cortijo, Singh, Liu, Medina-Morales, Yakah, Freedman and Martin2020). Studies on infant formulas supplemented with bovine MFGM have shown promising results on neurodevelopment (Timby et al., Reference Timby, Domellof, Hernell, Lonnerdal and Domellof2014) and defense against infections (Timby et al., Reference Timby, Hernell, Vaarala, Melin, Lönnerdal and Domellöf2015). Despite nutritional advances in infant formula with the addition of fragments of MFGM, commercial formulas still have shortcomings compared to human milk. For example, the configuration of the fat globule and the core triglyceride structure should be addressed in an integrated manner. The creation of a novel lipid system closer to human milk fat globule is thus an crucial step toward narrowing the gap between formula-fed and breastfed infants with regard to neurodevelopment, infectious diseases, and cholesterol metabolism.
Advances in infant nutrition
Research and innovation in the field of formulas for children aim not only to approximate the nutritional composition of breast milk, the aforementioned gold standard. The development of new infant formula will also provide benefits at a functional level, especially concerning protection against infectious diseases or metabolic disorders and the stimulation of immunological or neurocognitive development. Adding to molecular structure, the supramolecular structure of dietary lipids in human milk may also influence the bioavailability of PUFA for the developing brain. More recently, with the enhancement of knowledge regarding supramolecular structure's influence on the digestion and absorption of lipids (Gallier et al., Reference Gallier, Vocking, Post, Van De Heijning, Acton, Van Der Beek and Van Baalen2015), interest in simulating the globule structure of human milk fat in infant formula has increased (Wei et al., Reference Wei, Jin and Wang2019a).
The strategy of creating a droplet-based emulsion stable biomimetic human milk is a real technological change. The patented nutrition innovation Nuturis® is an infant formula developed with added milk fat globule membrane to a concept formula. The infant formula has large phospholipid coated lipid droplets (mode diameter 3–5 μm) that mimic the structure of lipids in human milk (Gallier et al., Reference Gallier, Vocking, Post, Van De Heijning, Acton, Van Der Beek and Van Baalen2015). The MFGM fractions are isolated from the by-products of the butter industry (buttermilk and butterserums: Conway et al., Reference Conway, Gauthier and Pouliot2014) using micro and ultrafiltration technologies. Another patented product is the Lacprodan® MFGM-10 designed with a unique protein and lipid profile, including compounds such as lactoferrin, IgG, sialic acid, phospholipids, and gangliosides. According to the manufacturer, Lacprodan® MFGM-10 results in significantly improved cognitive development among formula-fed infants at year one compared to a standard formula. Infants receiving formula supplemented with Lacprodan® MFGM-10 demonstrated similar cognitive performance as breastfed infants.
More than 3500 patents about infant formula were registered in an open database (espacenet patent) in the past five years. Among them, 105 targeted the use of MFGM isolates, 32 involved supplementation with bioactive components such as oligosaccharides and 141 invovled the use of the emulsion process using polar lipids (Espacenet Search, 2020). Besides the use of ingredients closer to human milk, different technological processes to produce new infant formula are also extensively mentioned in this database.
In order to mimic the TAG structure of human milk fat, lipid modification has been used and substitutes such as Betapol® and INFAT® were developed. These substitutes have the regiospecific distribution of the fatty acids similar to the positional distribution of the palmitic acid found in human milk. Both lipids are obtained after interesterification (enzymatic or chemical) (Innis, Reference Innis2011; Zou et al., Reference Zou, Pande and Akoh2016). These substitutes still are not yet seen as being used commonly in infant formulas, probably due to their cost.
The success of the human genome analysis and the initiative on precision medicine has accelerated progress towards personalized healthcare (Collins and Varmus, Reference Collins and Varmus2015). The use of omics technologies such as genomics, epigenomics, transcriptomics, proteomics, and metabolomics in health research has not only advanced the field of medicine but also revolutionized nutritional sciences (German, et al., Reference German, Zivkovic, Dallas and Smilowitz2011; Kellogg et al., Reference Kellogg, Dunn and Snyder2018). Food engineers, pediatricians, and nutritionists have more information to design infant formula for tailored needs and individuals to make personalized dietary decisions for health management.
Technological developments of MFGM
Several authors have reviewed the potential application of MFGM in dairy products and infant formula over the last decade (Singh, Reference Singh2006; Dewettinck et al., Reference Dewettinck, Rombaut, Thienpont, Le, Messens and Van Camp2008; Jiménez-Flores and Brisson, Reference Jiménez-Flores and Brisson2008; Spence et al., Reference Spence, Jimenez-Flores, Qian and Goddik2009). Although the physical-chemical characteristics and functions of the MFGM structure have not been completely elucidated, several technological methods designed to isolate MFGM or generate MFGM ingredients have been brought to the commercial-scale. As a result, the application of these ingredients as supplements for infant formula or other products is becoming increasingly practical.
There are many reasons why the dairy industry has not yet adopted a standard large-scale manufacturing process for MFGM ingredients. Native MFGM is very unstable during processing as handling of raw milk on farms, in transportation, in silos at manufacturing plants and during cream separation results in shear and fluid turbulence causing MFGM damage. Damage to MFGM may cause associated materials, including milk phospholipids, to be depleted from the native milk fat globules in the aqueous milk phase (Huang et al., Reference Huang, Zheng, Brennan, Mohan, Stipkovits, Li and Kulasiri2020). Consequently, more than half of the milk phospholipids in raw milk remain in skim milk (Rombaut et al., Reference Rombaut, Camp and Dewettinck2006; Britten et al., Reference Britten, Lamothe and Robitaille2008). Other limitations are the uncertainties and variables involved in the milk phospholipid fractionation processes.
The processes of isolating and purifying buttermilk MFGM components have been examined in several studies over the past decade (Astaire et al., Reference Astaire, Ward, German and Jiménez-Flores2003; Calvo et al., Reference Calvo, Martín-Hernández, García-Serrano, Castro-Gómez, Alonso-Miravalles, García-Martín, Megino-Tello, Alonso and Fontecha2020; Morin et al., Reference Morin, Pouliot and Jiménez-Flores2006; Reference Morin, Britten, Jiménez-Flores and Pouliot2007; Rombaut et al., Reference Rombaut, Camp and Dewettinck2006). For example, MFGM can be separated from fresh milk or dairy by-products such as buttermilk and butter serum, which are particularly rich in MFGM materials. Consequently, the isolation and proper use of MFGM properties can significantly increase the value-added element of dairy products. Depending on the raw cream's processing history, the nutritional and functional properties of the resulting buttermilk may vary considerably. Moreover, the origin (whey buttermilk, serum-butter or sweet buttermilk) of MFGM components and the matrix surrounding them both seem to affect the resultant biological activity. The MFGM fraction isolated from buttermilk can be utilized as a functional ingredient to stabilize oil-in-water emulsions. Recently, milk phospholipids isolated from MFGM fractions have become commercially available, opening up new possibilities based on phospholipid liposomes' ability to entrap bioactive compounds.
MFGM extracts can be obtained by combining physical processes, whereas with the extraction of specific phospholipids from MFGM-enriched fractions, solvent-extraction is the chosen method (Lee et al., Reference Lee, Kim, Hong, Suh and Jo2020; Price et al., Reference Price, Fei, Clark and Wang2018). Destabilization of the natural emulsion of the milk fat globule is the starting point of most commercial processes to produce MFGM-enriched ingredients (Huang et al., Reference Huang, Zheng, Brennan, Mohan, Stipkovits, Li and Kulasiri2020). For example, as illustrated in Fig. 5, the cream obtained by skimming whole milk constitutes the raw material for butter manufacturing. The churning of milk cream produces butter grains composed primarily of triacylglycerol (TAG) and buttermilk, as well as an aqueous phase containing MFGM constituents (Fig. 5). Buttermilk processing can involve evaporation and spray drying, however, in order to increase the protein and MFGM content of the powder, membrane separation processes can be applied. In cheesemaking processes, the cheese-whey is a by-product that is also considered to be a potential source of MFGM-enriched ingredients.

Fig. 5. Schematic representation of the different processing steps used in the manufacturing of butter and buttermilk.
MFGM-enriched compounds can also be concentrated by employing two other procedures, with the first one being the anhydrous milk fat production process. This process produces a >99.5% fat phase and an aqueous phase called beta serum that contains all MFGM components (Fontecha et al., Reference Fontecha, Brink, Wu, Pouliot, Visioli and Jiménez-Flores2020). The second method requires melting and holding butter at 60°C for 30 min, followed by centrifugal separation leading to a >99.5% fat oil phase and an aqueous buttermilk phase containing MFGM constituents (Fontecha et al., Reference Fontecha, Brink, Wu, Pouliot, Visioli and Jiménez-Flores2020).
Several attempts have been made to separate and isolate MFGM material from commercial buttermilk, with much of the focus on the separation of MFGM material from the proteins of the skim milk. The similarities in the size of casein micelles and MFGM components is the first major obstacle to overcome in order to achieve effective fractionation of buttermilk (Singh, Reference Singh2006). The extraction of MFGM components from buttermilk may include ultrafiltration, followed by supercritical fluid extraction to obtain an ingredient significantly enriched in phospholipids (Costa et al., Reference Costa, Elias-Argote, Jiménez-Flores and Gigante2010). By using micro-and ultrafiltration, the transmission of components through the membrane was found to depend on filtration conditions such as temperature, pH, pore size and type of membrane material and the type of buttermilk (Morin et al., Reference Morin, Pouliot and Jiménez-Flores2006; Rombaut et al., Reference Rombaut, Dejonckheere and Dewettinck2007). Sachdeva and Buchheim (Reference Sachdeva and Buchheim1997) proposed using rennet or the acidification to pH 4.6 following microfiltration in order to remove caseins before the concentration of MFGM. Using another approach, Corredig et al. (Reference Corredig, Roesch and Dalgleish2003) and Roesch et al. (Reference Roesch, Rincon and Corredig2004) used citrate to dissociate casein micelles, followed by microfiltration to collect MFGM material retentate. However, this application also causes loss of MFGM material (Rombaut et al., Reference Rombaut, Camp and Dewettinck2006). Moreover, the addition of a sodium citrate agent results in dispersion of not only casein micelles but also MFGM fragments (Rombaut et al., Reference Rombaut, Camp and Dewettinck2006). More recently Spitsberg et al. (Reference Spitsberg, Ivanov and Shritz2019) obtained considerable success in isolating MFGM from buttermilk using Ca-binding salts, but this was at a research level.
Price et al. (Reference Price, Fei, Clark and Wang2018) obtained different fractions of MFGM from whey protein concentrate using solvent extraction. Their study showed that different classes of phospholipids responded to ethanol concentration and extraction temperature differently. The optimum processing condition is dependent on the desired phospholipid content and recovery yield, and the highest phospholipid content was obtained using 70% ethanol at 70°C. For instance, sphingomyelin was favored during 70% ethanol extraction at 60°C, whereas phosphatidylserine enrichment required first removing other lipids.
The development of methods for the extraction of MFGM from buttermilk through microfiltration may increase the opportunity to produce this ingredient on a commercial scale. The conditions to which fat globules are exposed during processing affect the native structure and composition of MFGM and are likely to have consequences on its functionality. Hence, greater exploitation of the value of MFGM may require redesigning some of the dairy processes to which the globules are subjected.
In conclusion, the best nutrient source for infants continues to be breast milk from a healthy mother. However, under certain conditions, infant formulas must serve as either a sole or partial source of nutrition for at least the first year of life. Over the past few decades there has been a tremendous improvement in the nutritional quality of infant formulas. New ingredients are being developed that meet not only the infant's nutritional requirements but also provide other benefits such as immunity and enhanced cognitive development. Several additional health-related properties have been reported for MFGM, including anticholesterolemic effects, inhibition of cancer cell growth, and bactericidal properties. The MFGM structure is highly complex and composed of an assortment of lipids and proteins with specific nutritional end technological properties. Despite nutritional advances in infant formula with the addition of fragments of MFMG, commercial formulas still have shortcomings compared to human milk. The development of methods to isolate MFGM compounds from buttermilk should be expanded to produce this ingredient on a commercial scale. However, before the economics of such processes can be appreciated, the exceptional functionality of MFGM compounds needs to be further explored in order to be better understood.
Acknowledgements
Authors would like to acknowledge the support of the Agricultural Research Station at North Carolina Agricultural and Technical State University (Greensboro, NC 27411, USA). This research was funded, in part, by Grants (project Number NC.X337-5-21-170-1 and NC.X341-5-21-170-1) from the National Institute of Food and Agriculture (NIFA). Its contents are solely the responsibility of the authors and do not necessarily represent the official views of NIFA.