Introduction
This review is designed to investigate the exact cytoplasmic interactions which establish mammary lipid secretion as an apocrine process. This is generally held to be unique and remarkably uniform, morphologically, and proteomic studies across the seven mammalian genomes indicate a high conservation of genes apparently involved in milk fat globule secretion, which suggests that the cellular anatomy of secretion may be conserved across species (LeMay et al., Reference LeMay, Lynn and Martin2009).
There are two main scenarios: one which assumes that the observed close association between the cytoplasmic lipid droplets (CLD) and Golgi vesicles (GV) results in the CLD being released as a consequence of sequential exocytosis of the content of the associated GV. The second assumes that the CLD and the mammary epithelial cell (MEC) apical plasmalemma interact in some way which causes the CLD to rise out of the cytoplasm enveloped in the plasmalemma. This review presents the evidence for the two possibilities
Evolution
The mammary gland has a considerable palaeological history. Oftedal considers that a precursor predated the evolution of the true mammals. All investigators agree that it developed from an apocrine skin gland, initially to moisten and warm the fragile egg and latterly to provide nutrient support for the viviparous neonate (Blackburn et al., Reference Blackburn, Hayssen and Murphy1989; Oftedal, Reference Oftedal2012). The typical apocrine gland has two forms of secretion, one using exocytosis of GV content, and the other the release of particular apical pieces of the cell with no loss of cell viability.
Ultrastructural examination of a variety of present day apocrine skin glands reveals that the apical cell area to be released is defined by a collection of GVs at its base. Subsequent sequential exocytosis of these GVs releases the cell fragment while maintaining cell viability (Fig. 1: Smith and Hearn, Reference Smith and Hearn1979; Testa-Riva and Puxeddu, Reference Testa-Riva and Puxeddu1980; Gesase et al., Reference Gesase, Satoh and Ono1996). An evolutionary increase in size and development of branching of the gland would produce a protein rich secretion for neonatal support. The next evolutionary requirement is for an increase in nutrient value of the secretion which can readily be achieved by adding lipid to the secretion. Most epithelial cells have the enzymes necessary to produce triacylglyceride lipid droplets. The question is how to incorporate the lipid reliably into the apocrine secretory process. To investigate the evolution of this process there are only the details of present day mammary gland structure available, since there is no fossil evidence.
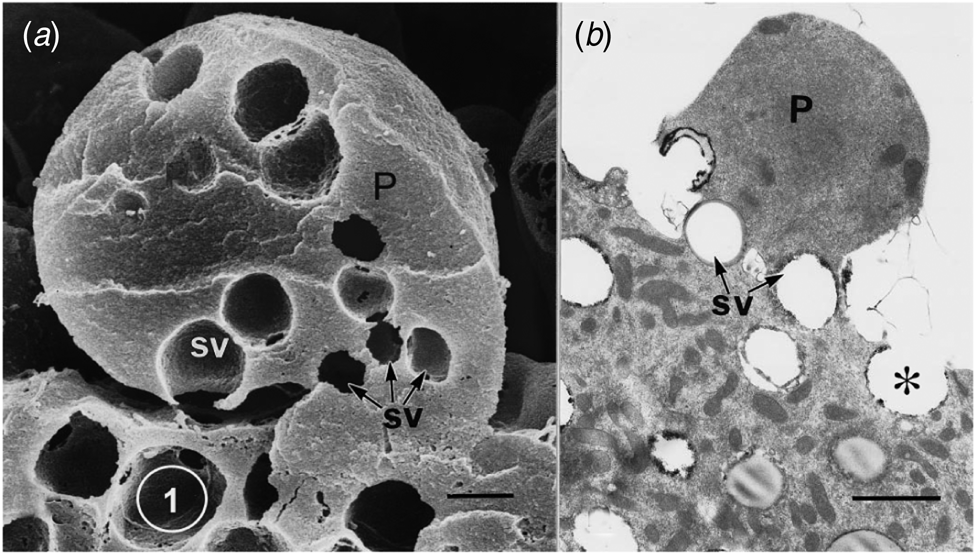
Fig. 1. Apical protrusions undergoing apocrine secretion (P). (a) Scanning electron micrograph showing secretory vacuoles (SV) at the base of the protrusion (P). Sequential exocytosis of the vesicles would release the protrusion. Bar: 1 μm. (b) Similar information is shown by the transmission electron micrograph. One secretory vesicle (asterisk) is exocytosing into the lumen of the gland. Scale bar: 2 μm. From Gesase et al. (Reference Gesase, Satoh and Ono1996). Courtesy of Springer Nature.
Milk fat globule (MFG) structure
Considering the variations in the structure of the mammalian placenta between the orders (Wooding and Burton, Reference Wooding and Burton2008), the morphological and biochemical similarities of the milk secretion process (the other half of the viviparous modification for reproductive success), is very surprising. This similarity also extends to the monotreme and marsupial milk secretion (Griffiths et al., Reference Griffiths, Elliott, Leckie and Schoefl1973). Consider the milk lipid secretion. In all species which have been examined at sufficient electron microscopic (EM) resolution the newly secreted spherical lipid MFG is closely surrounded by a unit membrane (UM: Bargmann and Knoop, Reference Bargmann and Knoop1959; Wooding, Reference Wooding1971a, Reference Wooding1971b, Reference Wooding2016; Freudenstein et al., Reference Freudenstein, Keenan, Eigel, Sasaki, Stadler and Franke1979). The UM is separated from the lipoprotein boundary present around what was the CLD by a uniform 10–15 nm layer derived from the cytoplasm. The cytoplasmic origin of this layer is demonstrated in the 3–8% of MFG which contain crescents of cytoplasm with recogniseable organelles (Wooding et al., Reference Wooding, Peaker and Linzell1970; Huston and Patton, Reference Huston and Patton1990). These crescents are continuous with the layer and confirm that the lipid secretion is apocrine. Crescents have been found in all milks so far investigated (Wooding et al., Reference Wooding, Peaker and Linzell1970; Huston and Patton, Reference Huston and Patton1990). How is such uniform packaging achieved?
MFG secretion
The non-lipid part of the milk (casein, lactose, calcium, water) is largely secreted by apical exocytosis of GV content. In the examples of skin gland apocrine secretion described above, the GV are also directly involved in cytoplasmic portion release and there is considerable evidence that this is also true for the mammary lipid secretion. Lipid droplets in the apical half of a well-fixed MEC in full lactation are usually closely associated with GV around their circumference (Figs. 2 and 3: Wooding, Reference Wooding1971a, Reference Wooding and Peaker1977). The GV are identified by their casein granule content and semiserial sectioning indicates that most if not all of the associated vesicles are from the Golgi. Recent light microscopy (LM) immunocytochemistry of MEC with casein antibodies confirms this (Fig. 4 and Wooding and Sargeant, Reference Wooding and Sargeant2015).

Fig. 2. Electron micrographs illustrating the Golgi vesicle–lipid droplet association (arrowheads) in mammary cells in full lactation. The position of the alveolus is indicated by an asterisk in all micrographs. (a) Sheep mammary gland. All of the apical lipid cytoplasmic lipid droplets (1–5) show associated Golgi vesicles (arrowheads). Golgi bodies and their vesicles (ga) are extensive; the vesicles are usually identified by their casein content. (b–d) Details of the Golgi vesicle – lipid association (arrowheads) in (b) sheep, (c) mouse and (d) cow. This illustrates the uniformity of the cytoplasmic gap between the vesicle membrane and the lipid contour, which is equivalent to the structure around the recently secreted alveolar milk fat globule (MFG), as seen in (g). (e–f) Rat mammary gland. (e) Typical Golgi vesicle–lipid association, showing distortion of the Golgi vesicles to follow the contour of the lipid (arrowheads). (f) Initiation of MFG secretion by exocytosis of a Golgi vesicle also associated with a cytoplasmic lipid droplet. (g) Horse mammary gland. High magnification of the structure of the recently secreted MFG membrane. (h) Sheep mammary gland. MFG nearly released, exocytosis of the remaining associated Golgi vesicles (arrowheads) at its base would continue and finally complete the process. Scale (a) 5 μm, (b–d, f) 200 nm, (e) 2 μm, (g) 50 nm, (h) 1 μm. Abbreviations: c, capillary; ga, golgi body; gv, golgi vesicle; L, lipid; n, nucleus of mammary cell; nm, nucleus of myoepithelial cell.
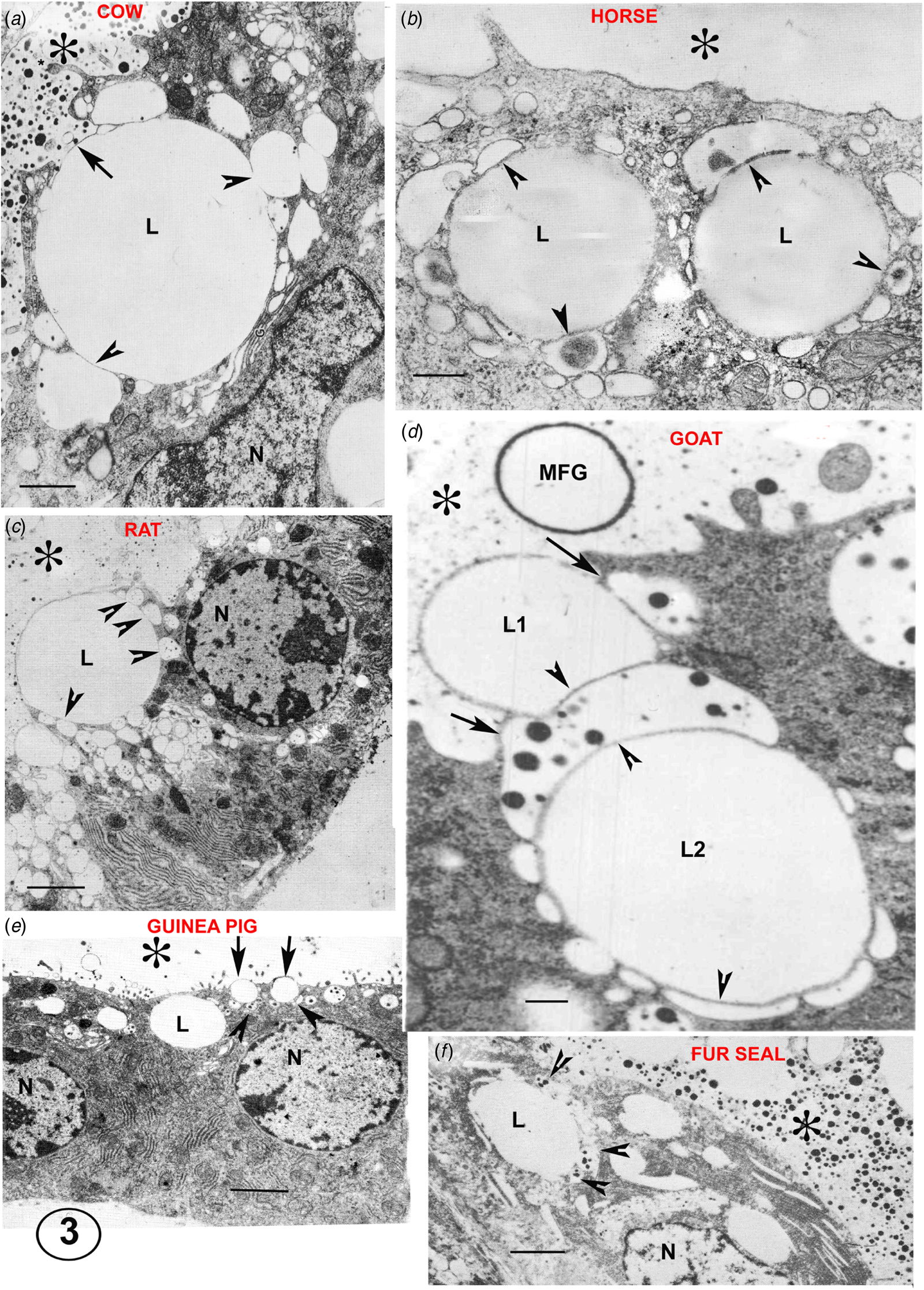
Fig. 3. Electron micrographs illustrating the Golgi vesicle–lipid droplet association (arrowheads) typical of mammary cells in full lactation in a variety of species. The position of the alveolus is indicated by an asterisk in all micrographs. In the cow (A), the arrow indicates the site of the first GV exocytosis initiating the process of MFG secretion. In the rat (C) the MFGM occupies one third of the secreting lipid (L) contour. In the guinea pig (E) the arrows indicate two small half secreted MFG with several tiny GV around at their bases in the cytoplasm (arrowheads). In the goat (D) exocytoses of the GV at the two arrows would probably release L1 as an MFG and initiate the secretion of L2. In the fur seal (F) despite the poor quality of the fixation lipid, GV associations are still visible (arrowheads). The fur seal produces milk with a lipid content close to 40% by volume but apparently uses the same secretion system. Scale bars all 2μm. Modified from Wooding (Reference Wooding and Peaker1977) and reproduced with permission of the Zoological Society of London.
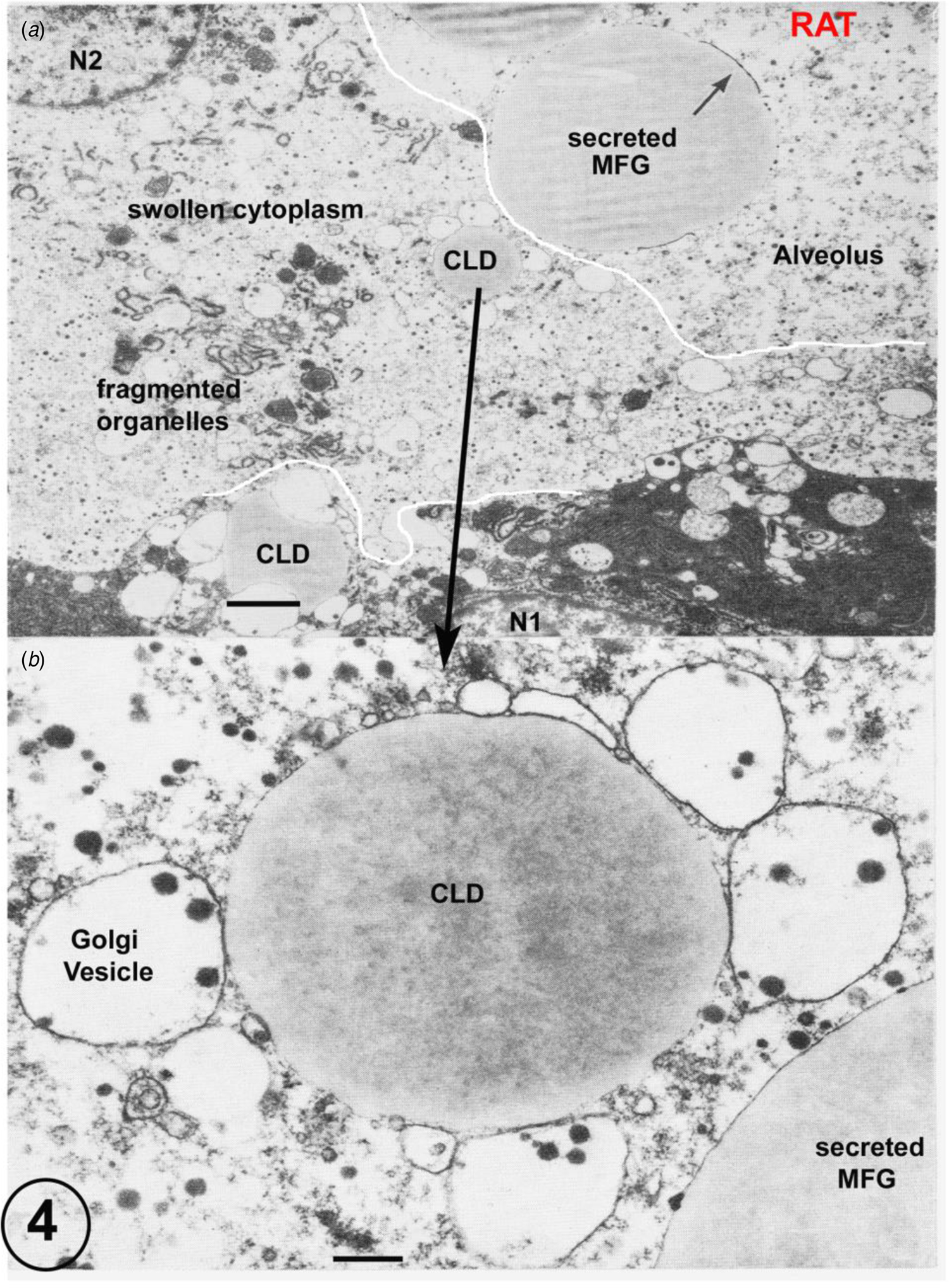
Fig. 4. (a) Rat lactating secretory epithelium fixed by immersion. Immersion fixation frequently produces considerably swollen cytoplasm and fragmented organelles together with a loss of the plasmalemma (predicted prefixation position indicated by the white line). This is clearly shown by the cell controlled by the nucleus N2, which also contains a cytoplasmic lipid droplet (CLD). This CLD, shown in detail in Figure B, has retained all of its apposed Golgi vesicles despite the disruption of the surrounding cytoplasm. The secreted MFG in the alveolus can be identified by the residual MFGM indicated by the arrow. The cell with nucleus N1 is much better fixed, but has still lost part of its plasmalemma (predicted prefixation position indicated by the white line). Scale Bars: (a) 5 μm; (b): 1 μm. Modified from Wooding (Reference Wooding and Peaker1977) and reproduced with permission of the Zoological Society of London.
This close association between lipid droplets and GV could be due to cytoplasmic crowding as the lipid droplets increase in volume. However, in immersion fixed material, which can produce a variable fixation quality, some cells show enormously artefactually expanded cytoplasm, but the GV remain closely associated with the lipid (Fig. 5). In addition, in tissue fixed well by perfusion, the membrane of the larger GV flattens close to the lipid contour (Figs. 2 and 3 and Wooding, Reference Wooding1971a, Reference Wooding and Peaker1977) but always separated by a 10–15 nm layer of cytoplasm, to produce an area equivalent to a mini MFG membrane (MFGM). As explained in more detail below, this association is probably based on interaction between a particular protein (adipophilin) on the lipid boundary and another transmembrane protein (butyrophilin) on the GV (see below and Fig. 6). If such a secretory vesicle was apical and firmly associated with the CLD, it is programmed (probably involving protein motors on microtubules: Rennison et al., Reference Rennison, Handel, Wilde and Burgoyne1992; Kjos et al., Reference Kjos, Vestre, Guadagno, Distefano and Progida2018) to travel up to, and then use attachment proteins (SNAP-receptor (SNARE) proteins where SNAP is soluble N-ethylmaleimide-sensitive factor attachment proteins: Chat et al., Reference Chat, Layania, Mahaut, Henry, Chanat and Truchet2011; Truchet et al., Reference Truchet, Chat and Ollivier-Bousquet2014; Honvo-Houéto et al., Reference Honvo-Houéto, Henry, Chat, Layania and Truchet2016) to fuse with the plasmalemma. The associated lipid droplet would ‘piggyback’ (Wooding, Reference Wooding2016) with the GV as a specific example of the more general system of cell organelle movements (‘hitchhiking’: Salogiannis and Reck-Peterson, Reference Salogiannis and Reck-Peterson2017). The GV fusion to the PM would then initiate the MFGM formation. Subsequent sequential exocytosis of the lateral CLD associated GV could gradually extend the MFGM with the elasticity of the cytoplasm causing the MFG to rise gradually out of the apical cytoplasm. EM images illustrating all stages of this secretion process can be seen in the literature in a variety of species (Wooding, Reference Wooding1971a, Reference Wooding and Peaker1977) and in Figures 2 and 3 in this review.

Fig. 5. Sheep mammary gland. Immunocytochemistry of butyrophilin and casein. Closely adjacent sections of the same alveolus (labelled with an asterisk and with different levels of the same capillary marked with a C. The 0.5–5-μm white circular areas in the cytoplasm of the mammary cells are lipid droplets, most obvious in (a). In the apical cytoplasm of cells in 4b (bovine butyrophilin), there are populations of even smaller white circular areas (double arrowheads) which by analogy with Figure 1a are most likely Golgi areas. This conclusion is reinforced by the fact that similar areas on Figures 5a (casein) and 4c (mouse butyrophilin) label positively. Arrows denote the same lipid droplet in at least two of the sections. Arrowheads in 4b and 4c indicate MFG in the process of secretion or an MFG free in the alveolus. The bovine butyrophilin antibody labels only the apical plasmalemma and alveolar MFG (arrowheads), whereas the mouse antibody labels cytoplasmic structures and the secreting and alveolar MFG but not the apical plasmalemma. Nuclei can occasionally be identified (curved arrow on 4a). Scale bar for all, 10 μm. From Wooding and Sargeant (Reference Wooding and Sargeant2015) courtesy of Sage Publishers.

Fig. 6. Diagram suggesting routes of milk fat globule (MFG) and crescent formation by sequential exocytoses of lipid associated Golgi vesicles (GV) with the plasmalemma (heterocytic fusion). The GV usually contain casein and express butyrophilin. Crescent formation may sometimes involve homocytic fusion between GV, which is rare, but has been shown in other contexts (see discussion of ‘presecretory vacuoles’ in the text and Fig. 7).
Protein involvement
It is critical to understand the basis for the attraction of the GV to the CLD and for the uniformity of the MFGM structure. This has been investigated using LM and EM structure and immunocytochemistry together with biochemical and proteomic studies. The problem with LM and EM is the static nature of any one micrograph, while the problem with the biochemical and proteomic studies is the difficulty in producing pure samples of apical MEC plasmalemma, GV or CLD.
It is generally now agreed that at least three proteins are involved (Mather and Keenan, Reference Mather and Keenan1998; Chong et al., Reference Chong, Reigan, Mayle-Combs, Orlicky and McManaman2011; Mather et al., Reference Mather, Masedunskas, Chen and Weigert2019; Monks et al., Reference Monks, Ladinsky and McManaman2020; Monks and Mather, Reference Monks and Mather2022). Firstly, a transmembrane butyrophilin1A1 (BTN) shown to be present in the MEC plasmalemma (Franke et al., Reference Franke, Heid, Grund, Winter, Freudenstein, Schmid, Jarasch and Keenan1981) and, more recently, on the GV (Fig. 4 and Wooding and Sargeant, Reference Wooding and Sargeant2015), which interacts with a second protein adipophilin (ADPH) (Chong et al., Reference Chong, Reigan, Mayle-Combs, Orlicky and McManaman2011, but now usually referred to as perilipin 2 or Plin 2, Mather et al., Reference Mather, Masedunskas, Chen and Weigert2019) which is firmly bound to the lipoprotein boundary of the CLD. This interaction is reinforced with a third cytoplasmic protein, xanthine oxidase (XO, also referred to as Xdh) and possibly other proteins such as the SNARE proteins which are directly involved in fusion of GV and PM and may also contribute to the close association between GV and CLD characteristic of full lactation (Truchet et al., Reference Truchet, Chat and Ollivier-Bousquet2014; Honvo-Houéto et al., Reference Honvo-Houéto, Henry, Chat, Layania and Truchet2016). Lateral association between the BTN, ADPH and XO is suggested to result in sheets of proteins producing the uniform separation between the plasmalemma or GV unit membranes and the lipoprotein boundary seen on well-fixed partially secreted EMs (Wooding, Reference Wooding1971a, Reference Wooding and Peaker1977). EM immunocytochemistry confirms that XO and BTN are present on sections of, and on isolated negatively stained, secondary MFGM preparations. After secretion of the MFG they form paracrystalline arrays in what was the cytoplasmic layer of the primary MFGM and these have been demonstrated in cow, human, goat and sheep secondary MFGMs (Wooding and Mather, Reference Wooding and Mather2017). The paracrystalline arrays are not seen until the continuous primary MFGM on a just-secreted MFG in the alveolus modifies to become discontinuous patches of primary MFGM. These lie on a continuous linear secondary MFGM based on the CLD boundary in the MEC (Wooding, Reference Wooding and Peaker1977; Bucheim, Reference Bucheim1982; Wooding and Mather, Reference Wooding and Mather2017).
BTN and XO and ADPH have been coopted by evolution for a vital role in MFG secretion from their previous immunologic and metabolic cellular functions. Ablation of any one of these genes severely disrupts MFG secretion (McManaman et al., Reference McManaman, Russell, Schaack, Orlicky and Robenek2007; Monks et al., Reference Monks, Orlicky, Libby, Dzieciatkowska, Ladinsky and McManaman2022). They are necessary to ensure that droplets are secreted in an efficient and regulated manner (Monks et al., Reference Monks, Dzieciatkowska, Bales, Orlicky, Wright and McManaman2016, Reference Monks, Ladinsky and McManaman2020, Reference Monks, Orlicky, Libby, Dzieciatkowska, Ladinsky and McManaman2022) with a minimal loss of MEC cytoplasm.
Secretion of the MFG is not necessarily continuous, the process may need to wait until enough exocytosis-prone butyrophilin carrying GV come into the correct association with the CLD. There is evidence from radioactive time course experiments (Stemberger and Patton, Reference Stemberger and Patton1981) and LM observations of living MEC that the secretion process takes hours (Masedunskas et al., Reference Masedunskas, Chen, Stussman, Weigert and Mather2017; Mather et al., Reference Mather, Masedunskas, Chen and Weigert2019). Constant GV trafficking to the apical surface will provide potential new membrane for the MFGM and final release of the MFG. Occasional homotypic GV fusions and exocytoses close to the forming MFG could also produce crescents of cytoplasmic material included within the secreted MFGM (Fig. 6).
The direct involvement of GV in the formation of the MFGM is also supported by the discovery that under certain non-physiological fixation conditions the exact structure of the MFGM can form around CLD in intracellular vacuoles by homotypic fusion of GV (Wooding, Reference Wooding1973 and for details see below).
The above scenario may be described as mammary vesicular lipid release and is based on evolutionary indications and uniform EM evidence of GV – CLD association from all mammalian species at full lactation so far investigated at sufficient resolution (Fig. 6).
Alternative theories
Most recent reviewers of MFG production suggest that inferring a secretory process from ‘static EMs’ is unconvincing and prefer the idea/description that the mammary CLD ‘become enveloped’ by the apical MEC plasmalemma as they ‘arise out of’, or ‘bud’ out of the cell (Mather and Keenan, Reference Mather and Keenan1998; Mather et al., Reference Mather, Masedunskas, Chen and Weigert2019; Monks et al., Reference Monks, Ladinsky and McManaman2020; Dai et al., Reference Dai, White, Liu and Liu2022; Monks and Mather, Reference Monks and Mather2022). There has been no suggestion as to where the energy required to achieve this comes from or how it is applied. If the ADPH primed CLD closely approached the BTN in the plasmalemma this would initiate interaction with XO and possibly other proteins. However, it seems biophysically very unlikely that this would provide sufficient force to pull a 1 to 10 μm CLD out of a viscous and crowded cytoplasm (Luby-Phelps, Reference Luby-Phelps2000). Nor is there any direct morphological or other evidence which indicates that CLDs use microtubule based motor proteins to push the CLD out of the MEC. No one has reported any evidence of actin based ‘pushing’ of the CLD out of the cytoplasm. There is also the problem of the actin scaffold immediately under the MEC apical plasmalemma clearly shown by recent examination of living mouse MEC (Mather et al., Reference Mather, Masedunskas, Chen and Weigert2019). A CLD with an apically-associated GV can penetrate such a scaffold using the microtubule motor system and SNARE proteins and other proteins which the GVs are programmed to use in order to navigate the cell (Rennison et al., Reference Rennison, Handel, Wilde and Burgoyne1992; Kjos et al., Reference Kjos, Vestre, Guadagno, Distefano and Progida2018). The apex of a CLD with no GV associated would have no such facilitator.
The concept of the MEC plasmalemma providing the only source of membrane for the MFG is historical. Bargmann and Knoop published the first EM evidence in 1959 showing a unit membrane, the plasmalemma (PM) around a half secreted MFG. It was then suggested that short range Van der Waal's molecular forces between the PM and the lipoprotein layer around the CLD might be sufficient to pull the CLD out of the cytoplasm (Patton and Fowkes, Reference Patton and Fowkes1967). However, later work showed that the distance between the layers was a constant 10–15 nm, far too great for the Van der Waals forces to be significant (Wooding, Reference Wooding1971a). The uniformity of the gap, and EM and biochemical studies of delipidated MFGM (Wooding and Kemp, Reference Wooding and Kemp1975), indicated intermolecular protein assemblies as suggested above.
There is also EM evidence that the exact structure of the MFGM can form from GV with no participation from the MEC plasmalemma (Fig. 7 and Wooding, Reference Wooding1973, Reference Wooding and Peaker1977) This has only been shown in goat mammary gland where the GV around the CLD homotypically fuse to form large intracellular vesicles containing MFG and casein granules. This is seen only in about 5% of the MEC in any one animal (Fig. 7, Wooding, Reference Wooding1973) and it must be emphasised that that this is not normal nor the basis for any significant secretion. It is a result of strange unpredictable fixation conditions in the cells which also produces swollen rough endoplasmic reticulum vesicles throughout the MEC, although the mitochondria and nuclei are well preserved (Fig. 7, Wooding, Reference Wooding1973). This unique homotypic intracellular GV fusion has only been observed in goats and not in any other species, but it does establish that the specific structure of the MFGM can be formed from GV without any participation of the MEC plasmalemma. Unfortunately, this evidence has been badly misinterpreted by reviewers (Mather and Keenan, Reference Mather and Keenan1998; Mather et al., Reference Mather, Masedunskas, Chen and Weigert2019; Monks and Mather, Reference Monks and Mather2022) as a normal physiological procedure. This was never suggested in the original paper (Wooding, Reference Wooding1973).
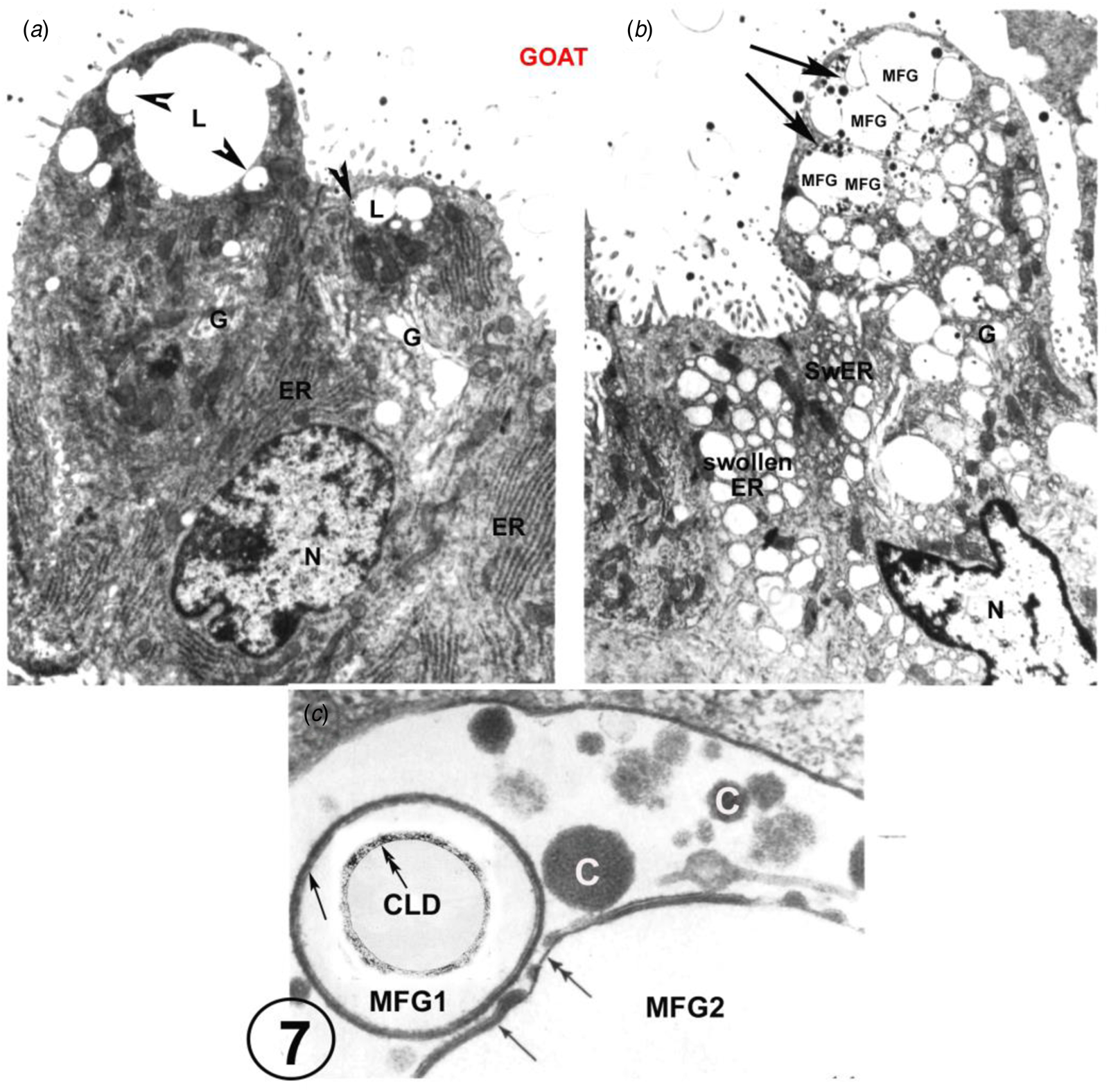
Fig. 7. Goat mammary secretory cells. A is from a normal perfusion fixed example, showing characteristic apical lipid droplets with associated Golgi vesicles (arrowheads), serried ranks of rough endoplasmic reticulum (ER) and an array of Golgi cisternae (G). In marked contrast, B shows swollen ER and Golgi as a result of unpredictable strange fixation conditions, and two ‘presecretory’ vacuoles (arrows). These vacuoles show casein granules and each contains two MFG, identified by the presence of enclosing MFGMs. C is a high magnification of part of a presecretory vacuole containing casein granules (c), and showing an MFG1 with an intact primary MFGM (single arrow) and part of MFG2 which has primary (single arrow) and secondary (double arrow) MFGM areas. Inset on MFG1 is a cytoplasmic lipid droplet (CLD) with a double arrow indicating the lipoprotein boundary. For details, zoom or see (Wooding, Reference Wooding1973). Scale bars: (a) and (b) 2 μm; (c) 200 nm. Modified from Wooding (Reference Wooding1973) and reproduced with permission from the Company of Biologists.
In the normal physiological situation, sequential rather than an instantaneous fusion of all the GV associated with the CLD would result in a gradual MFGM secretion process. EM images at all stages of such a secretory process can be found in the literature for a wide variety of species at full lactation (Figs. 2 and 3, Wooding, Reference Wooding1971a, Reference Wooding and Peaker1977). The current excellent and innovative intravital observations on mice (Mather et al., Reference Mather, Masedunskas, Chen and Weigert2019) have provided a clear indication of how the CLD form, move to and enlarge at the MEC apex. However, intravital evidence claiming that oxytocin is necessary for any release of MFG to the alveolus would make the process unique to the mouse. This is because of the considerable evidence that in denervated and/or transplanted mammary glands of goats, cows and guinea pigs no oxytocin is produced yet the glands produce the same amount of milk of the same composition of protein and lipid as normal (Linzell, Reference Linzell1963).
This means that the milk lipid is secreted into the alveolus with no oxytocin involvement. This fits well with a continuous vesicular secretion (as described above), which would be constitutive rather than requiring a secretogogue of some kind. The lack of any significant storage capacity in the mouse mammary gland might modify the lipid secretion mechanism, but the guinea pig has similar minimal storage but denervation (oxytocin elimination) does not reduce milk production.
It would be useful to examine the EM structure of the accumulated MFGs in the mouse mammary gland unsuckled for four hours to help resolve this disagreement. Also, the intravital claim that in mice ‘3-dimensional reconstructions of either intravital images or fixed tissue by confocal microscopy reveal that most lipid droplets that appear to have been secreted in 2-dimensional scans are still associated with cells below or above the plane of the section’ (Mather et al., Reference Mather, Masedunskas, Chen and Weigert2019) is clearly incompatible with my EM experience. In most if not all of the alveoli of the many mammary glands (including mice) I have examined with the EM there were many ‘free floating’ secreted droplets in the alveolar space unequivocally recogniseable by their secondary MFGM structure (Wooding, Reference Wooding1971b, Reference Wooding and Peaker1977; Wooding and Mather, Reference Wooding and Mather2017). This research also established that the packaging and release of the lipid in a minimum of cell constituents, the primary MFGM, was an elegant evolutionary solution to the need for the MEC to secrete large amounts of lipid while preserving its capacity for continued synthesis and secretion. Once the MFG is released into the alveolus the unit membrane immediately breaks down into characteristic patches (secondary MFGM) which show identical quasi crystalline arrays of BTN and ADPH in cow, goat, horse and human (Wooding and Mather, Reference Wooding and Mather2017 and as detailed above).
LM immunocytochemical demonstration that BTN is present in the Golgi lamellae and GV in goat, sheep and probably mouse (Wooding and Sargeant, Reference Wooding and Sargeant2015), provides the basis for the GV association with the CLD clearly shown on adequately fixed MEC in all species so far examined (Figs. 2–4, Wooding, Reference Wooding1971a, Reference Wooding and Peaker1977). This localisation is not too surprising since the BTN molecule has two N-linked mature highly branched oligosaccharide side chains (Sato et al., Reference Sato, Takio, Kobata, Greenwalt and Furukawa1995), whose synthesis would require Golgi processing (Strous, Reference Strous1986). However, this localisation has only been found using gold labelled rabbit-antimouse BTN peptide antibody on 1μm deplasticised LM sections. This technique (Groos et al., Reference Groos, Reale and Luciano2001) has been shown to confirm previous localisations and provides considerably better resolution and recognition of detail than fluorescent studies. On adjacent sections, lactoferrin and casein (both known to be processed via the Golgi and GV), demonstrate very similar localisations to buyrophilin (Wooding and Sargeant, Reference Wooding and Sargeant2015). Previous fluorescent immunocytochemistry, with both poly- and monoclonal antibodies to bovine or guinea-pig BTN on wax or frozen sections show a localisation only to the apical MEC plasmalemma (Franke et al., Reference Franke, Heid, Grund, Winter, Freudenstein, Schmid, Jarasch and Keenan1981; Jeong et al., Reference Jeong, Lisinski, Kadegowda, Shin, Wooding, Daniels, Schaack and Mather2013) which is also found on deplasticised sections (Fig. 4, Wooding and Sargeant, Reference Wooding and Sargeant2015). The differences in processing could well lead to differences in epitopes and/or epitope availability. A justification of the deplasticised section technique as well as the detailed evidence supporting the BTN GV localisation can be found in the Wooding and Sargeant (Reference Wooding and Sargeant2015) paper.
The MFGM will, therefore, consist largely of GV membrane. Even if there is a small contribution from the apical MEC plasmalemma during the MFG release process, such apical plasmalemma in the lactating MEC is likely to have originated as GV. It has recently been suggested that ‘it is obvious from the size and surface area of secretory vesicles and the volume of secreted skim milk that more vesicle membrane is added to the surface than is needed for the formation of the milk fat globule membrane’ (Monks and Mather, Reference Monks and Mather2022), so that the apical MEC plasmalemma may consist mainly of GV membrane at peak lactation secretion. However, individual lipid droplet secretion takes two to five hours as shown originally by Stemberger and Patton (Reference Stemberger and Patton1981) and confirmed by the recent intravital observations (Masedunskas et al., Reference Masedunskas, Chen, Stussman, Weigert and Mather1917). Thus, while the forming MFG unit membrane is bulging into the alveolus there is ample time for diffusion of typical plasmalemma specific or intracellular protein markers into that unit membrane.
Conclusion and further studies
The recent LM immunocytochemical demonstration of BTN in lactating MEC Golgi and vesicles considerably reinforces the ‘static EM’ evidence for the gradual GV based release of the MFG as being the major route for MFG secretion. There is no direct or other evidence for the process of ‘envelopment’ of the larger CLD by the PM of the MEC. Clearly, further work is necessary to resolve the many uncertainties and inconsistencies in the present views of mammary lipid secretion. High-resolution electron microscope immunocytochemistry on well-fixed lactating MECs would seem the best way of visualising any direct organellar interactions. Biochemical and proteomic studies, however detailed, on doubtfully ‘pure’ fractions of particular membranes can only indicate possibilities. For example, the suggestion that endoplasmic reticulum (ER) makes a ‘significant contribution to MFGM formation’ (Honvo-Houéto et al., Reference Honvo-Houéto, Henry, Chat, Layania and Truchet2016) has not been supported as yet by any EM evidence of ER associations with secreting MFG. In other cell types ER-PM membrane contact sites (MCS) have been established: the T-tubule – sarcoplasmic reticulum system in skeletal muscle is essential for controlling calcium secretion and consequent muscle contraction, for example. However, according to an excellent review by Phillips and Voeltz (Reference Phillips and Voeltz2016) there is no evidence for fusion of membranes at MCS sites which would seem to be the only way that the ER could ‘significantly contribute to MFGM formation’. These considerations emphasise the need to correlate the biochemical and proteomic results with the ‘static’ EMs if the detail of the MFG secretion process is to be clearly understood.
Acknowledgements
I am very grateful to Prof Malcolm Peaker for his encouragement and facilitation of this review summarising research we started so many years ago, initiated, of course, by Jim Linzell.