1. Introduction
The overall geological, magmatic and tectonometamorphic framework of the Attic-Cycladic crystalline belt (Fig. 1a) is well documented, but in detail there are still significant gaps in knowledge, particularly regarding geochronological aspects. One of these yet unresolved issues is the identification of tectonic slices within the Attic-Cycladic crystalline belt that have been affected by more than one high-pressure/low-temperature (HP/LT) metamorphic event (Gerogiannis et al. Reference Gerogiannis, Xypolias, Chatzaras, Aravadinou and Papapavlou2019). To address this point, we have studied blueschist-facies rocks from NW Andros (Figs 1, 2) where the metamorphic succession comprises two tectonic subunits, the Makrotantalon Unit and the Lower Unit (Papanikolaou, Reference Papanikolaou1978 a,b). On the basis of mineralogical and geochronological evidence, the Lower Unit of Andros can be correlated with the Cycladic blueschist sequences (= Cycladic Blueschist Unit), which underwent eclogite- to epidote-blueschist-facies metamorphism and subsequent overprinting at lower P–T conditions between c. 55 Ma and c. 12 Ma (e.g. Okrusch & Bröcker Reference Okrusch and Bröcker1990; Wijbrans et al. Reference Wijbrans, Schliestedt and York1990; Bröcker et al. Reference Bröcker, Kreuzer, Matthews and Okrusch1993, Reference Bröcker, Baldwin and Arkudas2013; Tomaschek et al. Reference Tomaschek, Kennedy, Villa, Lagos and Ballhaus2003; Lagos et al. Reference Lagos, Scherer, Tomaschek, Münker, Keiter, Berndt and Ballhaus2007; Ring et al. Reference Ring, Glodny, Will and Thomson2010; Cliff et al. Reference Cliff, Bond, Butler and Dixon2017; Peillod et al. Reference Peillod, Ring, Glodny and Skelton2017; Laurent et al. Reference Laurent, Huet, Labrousse, Jolivet, Monie and Augier2017, Reference Laurent, Lanari, Naïr, Augier, Lahfid and Jolivet2018; Lamont et al. Reference Lamont, Searle, Gopon, Roberts, Wade, Palin and Waters2020 b; Glodny & Ring, Reference Glodny and Ring2022).
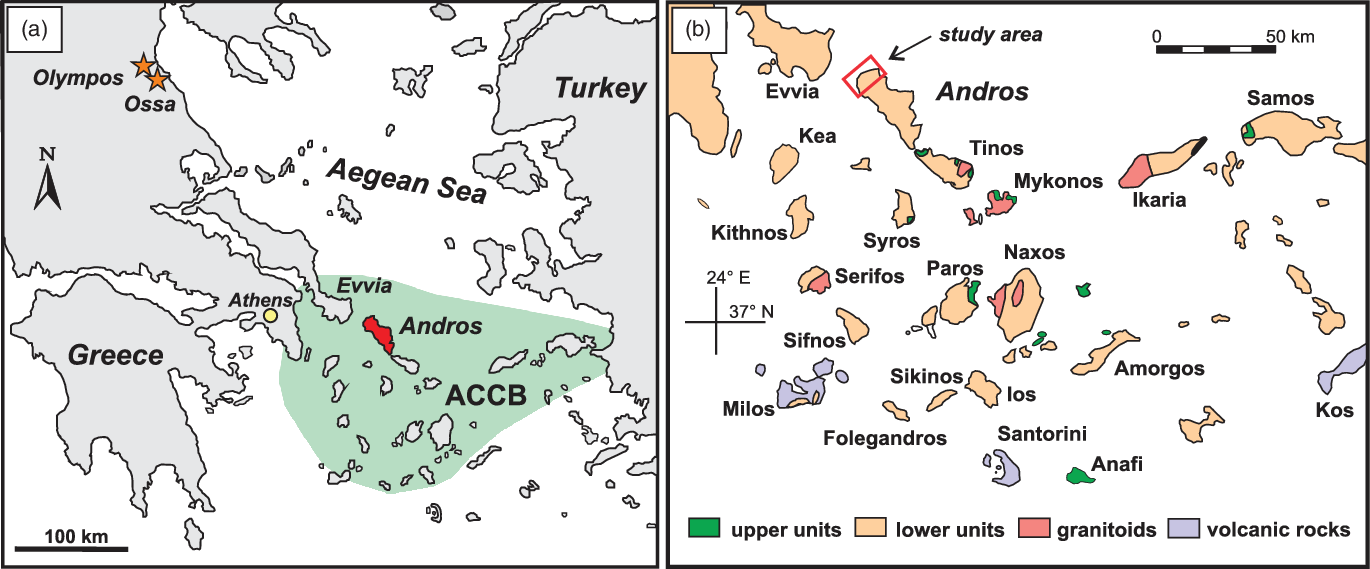
Fig. 1. (a) Geographical overview of the larger study area. ACCB – Attic-Cycladic crystalline belt; stars indicate approximate locations of the Olympos and Ossa tectonic windows. (b) Close-up of the ACCB with schematic overview of the main geological units (modified after Matthews & Schliestedt, Reference Matthews and Schliestedt1984). Red rectangle indicates location of the study area that is shown in more detail in Figure 2.
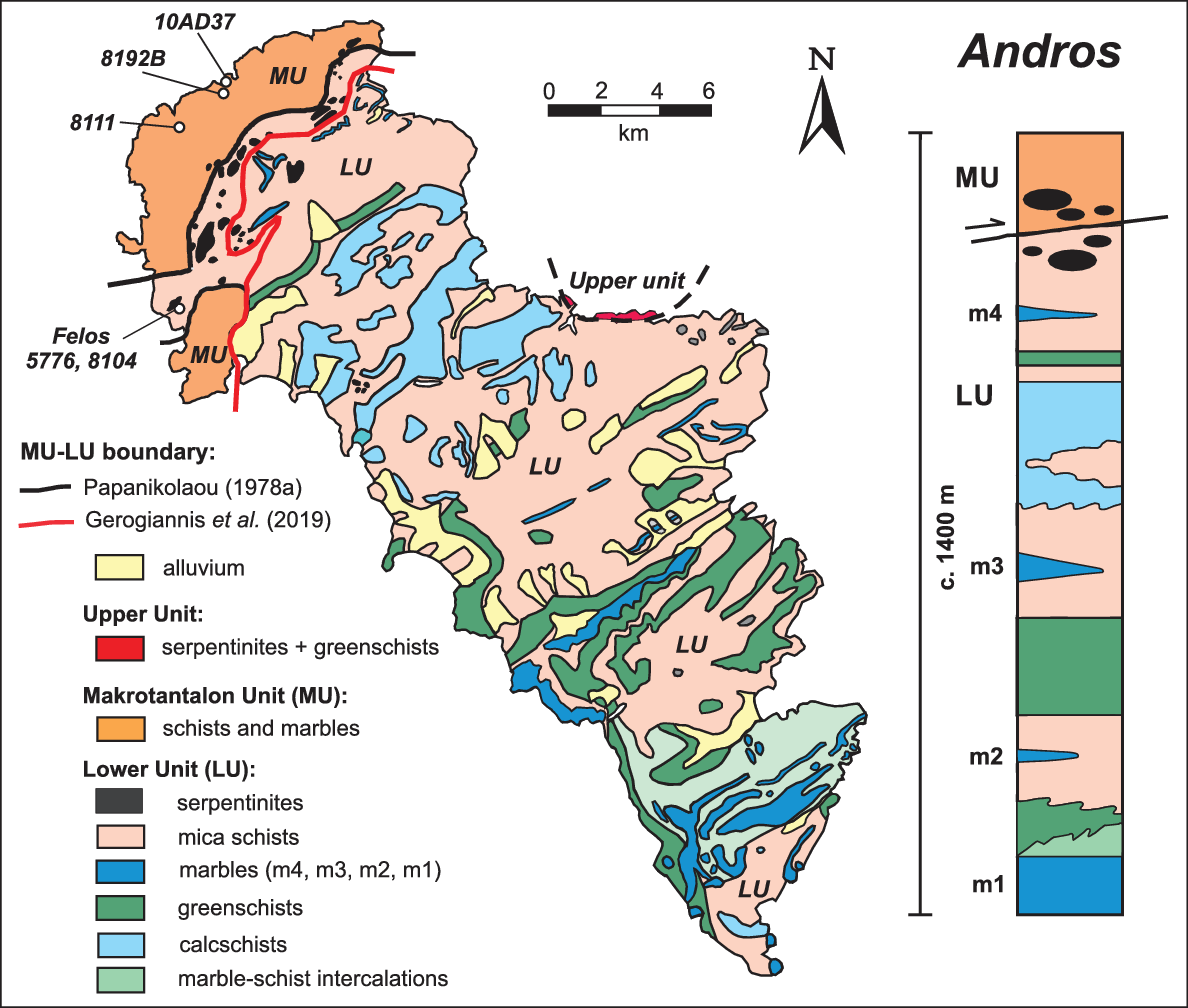
Fig. 2. Simplified geological map and columnar section of Andros (modified after Papanikolaou, Reference Papanikolaou1978 a).
The status of the Makrotantalon Unit within the structural framework of the Attic-Cycladic crystalline belt is controversial, with some studies suggesting a relationship to the Cycladic HP/LT sequences (e.g. Papanikolaou, Reference Papanikolaou1978 b, Reference Papanikolaou and Helgeson1987; Shaked et al. Reference Shaked, Avigad and Garfunkel2000; Huyskens & Bröcker, Reference Huyskens and Bröcker2014; Gerogiannis et al. Reference Gerogiannis, Xypolias, Chatzaras, Aravadinou and Papapavlou2019), while others assume that the Makrotantalon Unit belongs to the Upper Unit (Dürr, Reference Dürr and Jacobshagen1986; Bröcker & Franz, Reference Bröcker and Franz2006; Huet et al. Reference Huet, Labrousse, Monié, Malvoisin and Jolivet2015), or represents an intermediate position between the main units (Mehl et al. Reference Mehl, Jolivet, Lacombe, Labrousse, Rimmele, Taymaz, Yilmaz and Dilek2007). Major arguments for a correlation with the Upper Unit were the tectonic position on top of the Lower Unit, the presumed lack of HP/LT metamorphism and the Cretaceous dates of greenschist-facies rocks (Bröcker & Franz, Reference Bröcker and Franz2006; Huyskens & Bröcker, Reference Huyskens and Bröcker2014), which are unknown from the structurally lower sequences but are typical for low- to medium-grade metamorphic rocks and granitoids on top of the Cycladic Blueschist Unit (e.g. Patzak et al. Reference Patzak, Okrusch and Kreuzer1994; Martha et al. Reference Martha, Dörr, Gerdes, Petschick, Schastok, Xypolias and Zulauf2016).
Unambiguous evidence for blueschist-facies conditions in the Makrotantalon Unit was interpreted as confirmation of a relationship with the Cycladic Blueschist Unit (Huyskens & Bröcker, Reference Huyskens and Bröcker2014). This conclusion was questioned by Huet et al. (Reference Huet, Labrousse, Monié, Malvoisin and Jolivet2015) who reported 40Ar–39Ar phengite dates of ∼116 Ma for a glaucophane-garnet schist from the basal part of the Makrotantalon Unit (Fig. 2). Early Cretaceous HP/LT metamorphism has not been observed in the typical Cycladic blueschist sequences, but does occur in the Pelagonian Zone of mainland Greece (e.g. Schermer, Reference Schermer1990; Schermer et al. Reference Schermer, Lux and Burchfiel1990; Lips et al. Reference Lips, White and Wijbrans1998, Reference Lips, Wijbrans, White, Durand, Jolivet, Horvàth and Séranne1999). Accordingly, Huet et al. (Reference Huet, Labrousse, Monié, Malvoisin and Jolivet2015) suggested a Pelagonian affinity for the Makrotantalon Unit as also indicated by the Cretaceous Rb–Sr dates (∼100–90 Ma and ∼80–70 Ma) of greenschist-facies rocks from this tectonic unit (Bröcker & Franz, Reference Bröcker and Franz2006; Huyskens & Bröcker, Reference Huyskens and Bröcker2014).
Detailed geological and structural mapping at a scale of 1:15 000 led Gerogiannis et al. (Reference Gerogiannis, Xypolias, Chatzaras, Aravadinou and Papapavlou2019) to reinterpret the position of the tectonic contact between the Makrotantalon Unit and the Lower Unit and thus the structural position of previously dated samples. On the basis of the new map, Gerogiannis et al. (Reference Gerogiannis, Xypolias, Chatzaras, Aravadinou and Papapavlou2019) inferred that an epidote-glaucophane schist with a Rb–Sr date of ∼45 Ma, originally assigned to the Lower Unit (Bröcker & Franz, Reference Bröcker and Franz2006), as well as greenschist-facies samples with similar apparent ages from other parts of NW Andros (Huyskens & Bröcker, Reference Huyskens and Bröcker2014), belong instead to the Makrotantalon Unit. The revised geological map implies that both Cretaceous and Eocene blueschist-facies rocks are preserved within a distinct subunit of the Cycladic Blueschist Unit. However, this interpretation is still fraught with uncertainty as it is essentially based on the structural assignment of samples collected near a tectonic contact that is challenging to localize in the field owing to the lack of a well-defined shear zone (Mehl et al. Reference Mehl, Jolivet, Lacombe, Labrousse, Rimmele, Taymaz, Yilmaz and Dilek2007; Huyskens & Bröcker, Reference Huyskens and Bröcker2014). This makes the assignment of samples to the hanging and footwall extremely difficult. The apparent coexistence of blueschist-facies rocks with different ages also raises the question of whether the accuracy of the older dates is compromised by extraneous Ar (e.g. Kelley, Reference Kelley2002; Laurent et al. Reference Laurent, Huet, Labrousse, Jolivet, Monie and Augier2017).
This study aims to substantiate the geological significance of the Cretaceous 40Ar–39Ar dates (Huet et al. Reference Huet, Labrousse, Monié, Malvoisin and Jolivet2015) and to clarify the presumed status of the Makrotantalon Unit as a nappe with Pelagonian origin in the Cycladic Blueschist Unit (Huyskens & Bröcker, Reference Huyskens and Bröcker2014; Gerogiannis et al. Reference Gerogiannis, Xypolias, Chatzaras, Aravadinou and Papapavlou2019). To achieve these goals, we have dated blueschist-facies rocks from various locations in NW Andros using Rb–Sr geochronology, including two samples from the previously 40Ar–39Ar-dated blueschist area and three samples collected at a greater distance from the tectonic contact in other parts of the Makrotantalon Unit. Using new and published metamorphic ages, the following questions are addressed: Is the Makrotantalon Unit a tectonic slice of the Cycladic Blueschist Unit that records pre-Eocene blueschist metamorphism? Are the Cretaceous 40Ar–39Ar dates of blueschists from the Makrotantalon Unit compromised by either inherited or excess Ar? Did the Makrotantalon Unit undergo separate episodes of HP/LT metamorphism in Cretaceous and Eocene times?
2. Geological setting
The Attic-Cycladic crystalline belt comprises two major tectonic units with different pressure–temperature (P–T), time and deformation histories (e.g. Dürr et al. Reference Dürr, Altherr, Keller, Okrusch, Seidel, Closs, Roeder and Schmidt1978; Dürr, Reference Dürr and Jacobshagen1986; Okrusch & Bröcker, Reference Okrusch and Bröcker1990; Ring et al. Reference Ring, Glodny, Will and Thomson2010). The structurally higher unit (Upper Unit) is poorly preserved and includes a heterogeneous sequence of unmetamorphosed sediments, Permian to Mesozoic metasediments, Jurassic ophiolites, ophiolites of unknown age, Cretaceous to Palaeogene greenschist-facies rocks, as well as Late Cretaceous granitoids, amphibolites and gneisses (e.g. Patzak et al. Reference Patzak, Okrusch and Kreuzer1994; Martha et al. Reference Martha, Dörr, Gerdes, Petschick, Schastok, Xypolias and Zulauf2016; Lamont et al. Reference Lamont, Roberts, Searle, Gopon, Waters and Millar2020 a). The Upper Unit lacks evidence for HP/LT metamorphism, which is a key feature of the structurally lower sequences (= Cycladic Blueschist Unit), but its metamorphic rocks could still record subduction-related processes that occurred at a slightly earlier date and at different P–T conditions compared to the Cycladic Blueschist Unit (Lamont et al. Reference Lamont, Roberts, Searle, Gopon, Waters and Millar2020 a).
The Cycladic Blueschist Unit consists of a pre-Alpine crystalline basement and a stack of tectonic units comprising a metamorphosed volcano-sedimentary succession with a polymetamorphic evolution, including an eclogite- to epidote-blueschist-facies event and a greenschist- to upper-amphibolite-facies overprint (e.g. Dürr et al. Reference Dürr, Altherr, Keller, Okrusch, Seidel, Closs, Roeder and Schmidt1978; Dürr, Reference Dürr and Jacobshagen1986; Papanikolaou, Reference Papanikolaou and Helgeson1987; Okrusch & Bröcker Reference Okrusch and Bröcker1990; Ring et al. Reference Ring, Glodny, Will and Thomson2010; Philippon et al. Reference Philippon, Brun and Gueydan2012; Glodny & Ring, Reference Glodny and Ring2022). Various geochronological methods, including white mica (K–Ar, Ar–Ar, Rb–Sr), zircon (U–Pb) and garnet (Sm–Nd, Lu–Hf) dating, indicated Eocene (c. 55 Ma) to Miocene (c. 12 Ma) ages that were interpreted to date HP/LT metamorphism and several stages of lower pressure overprinting, respectively (e.g. Altherr et al. Reference Altherr, Schliestedt, Okrusch, Seidel, Kreuzer, Harre, Lenz, Wendt and Wagner1979, Reference Altherr, Kreuzer, Wendt, Lenz, Wagner, Keller, Harre and Höhndorf1982; Wijbrans et al. Reference Wijbrans, Schliestedt and York1990; Bröcker et al. Reference Bröcker, Kreuzer, Matthews and Okrusch1993; Tomaschek et al. Reference Tomaschek, Kennedy, Villa, Lagos and Ballhaus2003; Forster & Lister, Reference Forster and Lister2005; Putlitz et al. Reference Putlitz, Cosca and Schumacher2005; Lagos et al. Reference Lagos, Scherer, Tomaschek, Münker, Keiter, Berndt and Ballhaus2007; Bröcker et al. Reference Bröcker, Baldwin and Arkudas2013; Dragovic et al. Reference Dragovic, Baxter and Caddick2015; Cliff et al. Reference Cliff, Bond, Butler and Dixon2017; Laurent et al. Reference Laurent, Huet, Labrousse, Jolivet, Monie and Augier2017; Peillod et al. Reference Peillod, Ring, Glodny and Skelton2017). So far, interpretations suggesting a prolonged Cretaceous–Eocene subduction and exhumation history that are based on U–Pb zircon ages (Bröcker & Enders, Reference Bröcker and Enders1999; Bröcker & Keasling, Reference Bröcker and Keasling2006) could not be unambiguously confirmed and have been attributed to erroneous linking of protolith ages to metamorphic processes (e.g. Fu et al. Reference Fu, Valley, Kita, Spicuzza, Paton, Tsujimori, Bröcker and Harlow2010; Bulle et al. Reference Bulle, Bröcker, Gärtner and Keasling2010). However, metamorphic sole ages of the Tsiknias ophiolite on Tinos also suggest a pre-Eocene stage of subduction and ophiolite obduction at c. 74–66 Ma (Lamont et al. Reference Lamont, Roberts, Searle, Gopon, Waters and Millar2020 a).
On Andros, the metamorphic succession can be subdivided into three tectonic units, the Lower Unit, the Makrotantalon Unit and the Upper Unit (Papanikolaou, Reference Papanikolaou1978 a,b; Mehl et al. Reference Mehl, Jolivet, Lacombe, Labrousse, Rimmele, Taymaz, Yilmaz and Dilek2007; Gerogiannis et al. Reference Gerogiannis, Xypolias, Chatzaras, Aravadinou and Papapavlou2019). The Upper Unit only occurs in small outcrops at the NE coast of the island and mainly consists of greenschists and serpentinites, which are separated from the Lower Unit by a flat-lying detachment (Mehl et al. Reference Mehl, Jolivet, Lacombe, Labrousse, Rimmele, Taymaz, Yilmaz and Dilek2007). The Lower Unit (up to 1200 m thick) is correlative with the Cycladic blueschist sequences that build up most parts of the neighbouring islands (Syros, Sifnos, Tinos). The main rock types are clastic metasediments, carbonate-rich schists, marbles and various metavolcanic rocks (Papanikolaou, Reference Papanikolaou1978 a,b; Gerogiannis et al. Reference Gerogiannis, Xypolias, Chatzaras, Aravadinou and Papapavlou2019), which locally are intercalated with manganese-rich quartzites and schists (Reinecke, Reference Reinecke1986; Reinecke et al. Reference Reinecke, Okrusch and Richter1985). Ion probe U–Pb zircon dating of felsic metavolcanic rocks yielded Triassic protolith ages (∼249–240 Ma; Bröcker & Pidgeon, Reference Bröcker and Pidgeon2007). Most lithologic units are characterized by greenschist-facies mineral assemblages, but glaucophane-bearing rocks are sporadically preserved (e.g. Papanikolaou, Reference Papanikolaou1978 a,b; Mehl et al. Reference Mehl, Jolivet, Lacombe, Labrousse, Rimmele, Taymaz, Yilmaz and Dilek2007). Strongly overprinted rocks mostly yielded Miocene Rb–Sr dates (c. 23–21 Ma), whereas Eocene Rb–Sr dates (c. 44–39 Ma) were reported for the HP/LT event (Bröcker & Franz, Reference Bröcker and Franz2006; Huyskens & Bröcker, Reference Huyskens and Bröcker2014). Estimates of metamorphic conditions indicate temperatures of 450–500 °C and a minimum pressure of 1 GPa for the HP/LT stage, whereas the greenschist-facies overprint occurred at 350–520 °C and 0.5–0.9 GPa (Reinecke, Reference Reinecke1986; Bröcker & Franz, Reference Bröcker and Franz2006).
The Makrotantalon Unit structurally overlies the Lower Unit and is only exposed in the northern part of the island (Fig. 2). The exact position of the inferred tectonic contact between the units is difficult to localize mainly because of intense overprinting by exhumation-related structures (e.g. Papanikolaou, Reference Papanikolaou1978 b; Mukhin, Reference Mukhin1996; Mehl et al. Reference Mehl, Jolivet, Lacombe, Labrousse, Rimmele, Taymaz, Yilmaz and Dilek2007; Huyskens & Bröcker, Reference Huyskens and Bröcker2014; Gerogiannis et al. Reference Gerogiannis, Xypolias, Chatzaras, Aravadinou and Papapavlou2019). Huyskens & Bröcker (Reference Huyskens and Bröcker2014) considered a discontinuous serpentinite belt that extends through NW Andros (Papanikolaou, Reference Papanikolaou1978 a,b) as a suitable marker of the shear zone, but a clear assignment of the ultramafic rocks to one of the two nappes is difficult. From lithostratigraphic observations, Papanikolaou (Reference Papanikolaou1978 b) interpreted the ultramafic rocks as a distinct olistostromatic horizon within the Lower Unit, placing the tectonic contact above the serpentinite belt. T. A. Shin (unpub. Master’s thesis, Univ. Texas at Austin, 2014) suggested that the serpentinites are part of the Makrotantalon Unit, inferring a tectonic contact below the ultramafic rocks. In contrast, Gerogiannis et al. (Reference Gerogiannis, Xypolias, Chatzaras, Aravadinou and Papapavlou2019) concluded that the serpentinite bodies are exposed at different structural levels within both tectonic units and thus cannot be used for delineating the nappe contact. These authors used lithological differences and the presence of mylonitic rocks for mapping of the boundary between the units. Eocene dates (∼40 Ma; Rb–Sr internal isochrons) recorded in samples collected close to the presumed shear zone were interpreted as the lower time limit for tectonic juxtaposition (Huyskens & Bröcker, Reference Huyskens and Bröcker2014). Permian fossils in dolomitic marbles of the Makrotantalon Unit and Triassic or younger zircon protolith or maximum sedimentation ages of the Lower Unit support the interpretation that the units were originally separated by a thrust zone (Papanikolaou, Reference Papanikolaou1978 a,b; Huyskens & Bröcker, Reference Huyskens and Bröcker2014). Other studies suggested the existence of a presumably low-angle normal fault with top-to-the-NE kinematics, proposed the reactivation of an earlier thrust fault as a normal fault or questioned whether a tectonic contact exists at all (Dürr, Reference Dürr and Jacobshagen1986; Avigad & Garfunkel, Reference Avigad and Garfunkel1991; Avigad et al. Reference Avigad, Garfunkel, Jolivet and Azañon1997; Bröcker & Franz, Reference Bröcker and Franz2006; Bröcker & Pidgeon, Reference Bröcker and Pidgeon2007; Mehl et al. Reference Mehl, Jolivet, Lacombe, Labrousse, Rimmele, Taymaz, Yilmaz and Dilek2007; Huyskens & Bröcker, Reference Huyskens and Bröcker2014; Huet et al. Reference Huet, Labrousse, Monié, Malvoisin and Jolivet2015). Gerogiannis et al. (Reference Gerogiannis, Xypolias, Chatzaras, Aravadinou and Papapavlou2019) found no structural evidence for reactivation of a thrust as a low-angle normal fault but inferred that the original contact was folded during exhumation and transposed by NE-directed shearing. Gerogiannis et al. (Reference Gerogiannis, Xypolias, Chatzaras, Aravadinou and Papapavlou2019) also reasoned that structural data from Andros are more consistent with NE- than with SW-directed ductile extrusion, as suggested for other parts of the Cyclades (e.g. Doutsos et al. Reference Doutsos, Pe-Piper, Boronkay and Koukouvelas1993; Xypolias et al. Reference Xypolias, Kokkalas and Skourlis2003, Reference Xypolias, Iliopoulos, Chatzaras and Kokkalas2012; Aravadinou et al. Reference Aravadinou, Xypolias, Chatzaras, Iliopoulos and Gerogiannis2016; Gerogiannis & Xypolias, Reference Gerogiannis and Xypolias2017).
The Makrotantalon Unit (up to 600 m thick) mainly consists of clastic metasedimentary rocks, dolomite marbles and minor metabasic schists (Papanikolaou, Reference Papanikolaou1978 a,b; Gerogiannis et al. Reference Gerogiannis, Xypolias, Chatzaras, Aravadinou and Papapavlou2019). Glaucophane-bearing rocks are locally preserved, but greenschist-facies mineral assemblages are more common (Mukhin, Reference Mukhin1996; Huyskens & Bröcker, Reference Huyskens and Bröcker2014; Gerogiannis et al. Reference Gerogiannis, Xypolias, Chatzaras, Aravadinou and Papapavlou2019). For blueschist-facies rocks, multi-equilibrium thermobarometry indicates peak metamorphic conditions of 550 °C and 1.85 GPa (Huet et al. Reference Huet, Labrousse, Monié, Malvoisin and Jolivet2015), which are largely consistent with P–T estimates for Eocene HP/LT rocks of the Cycladic Blueschist Unit on neighbouring islands (e.g. Parra et al. Reference Parra, Vidal and Jolivet2002; Philippon et al. Reference Philippon, Brun and Gueydan2012; Laurent et al. Reference Laurent, Lanari, Naïr, Augier, Lahfid and Jolivet2018; Lamont et al. Reference Lamont, Searle, Gopon, Roberts, Wade, Palin and Waters2020 b; Glodny & Ring, Reference Glodny and Ring2022 and references therein). Greenschist-facies rocks of the Makrotantalon Unit record P–T conditions of 350–455 °C and 0.41–0.54 GPa (Bröcker & Franz, Reference Bröcker and Franz2006). Single phengite grains of a glaucophane-garnet schist from the basal part of the Makrotantalon Unit yielded 40Ar–39Ar plateau dates of ∼116 Ma. White mica geochronology of greenschist-facies rocks produced Rb–Sr dates between c. 104 Ma and c. 21 Ma, which were interpreted to document distinct metamorphic episodes in the Cretaceous (c. 100–90 Ma and c. 80–70 Ma) and Miocene (c. 24–21 Ma) periods (Bröcker & Franz, Reference Bröcker and Franz2006). The new map by Gerogiannis et al. (Reference Gerogiannis, Xypolias, Chatzaras, Aravadinou and Papapavlou2019) indicates that a HP/LT rock with a Rb–Sr date of c. 45 Ma, originally interpreted as part of the Lower Unit (Bröcker & Franz, Reference Bröcker and Franz2006), was instead collected in the Makrotantalon Unit, suggesting an even more complex polymetamorphic history.
On a regional scale, the Makrotantalon Unit can be correlated with the HP/LT nappe stack exposed in southern Evia where the Cycladic Blueschist Unit comprises three subunits (Ochi, Styra and Tsaki; e.g. Papanikolaou, Reference Papanikolaou1978 b; Katzir et al. Reference Katzir, Avigad, Matthews, Garfunkel and Evans2000; Shaked et al. Reference Shaked, Avigad and Garfunkel2000). Gerogiannis et al. (Reference Gerogiannis, Xypolias, Chatzaras, Aravadinou and Papapavlou2019) interpreted the Makrotantalon Unit as the lateral equivalent of the Ochi nappe on Evia (as also suggested by Papanikolaou, Reference Papanikolaou2013), because of its identical structural position, lithological similarities and matching tectonometamorphic history. In contrast, Huyskens & Bröcker (Reference Huyskens and Bröcker2014) questioned a clear relationship to a specific tectonic slice on Evia and considered the possibility that the Makrotantalon Unit may represent a distinct subunit with similarities to the tectonic-metamorphic history of the south Evia nappe stack.
3. Methods
Mineral compositions were determined with a JEOL 8530F electron microprobe (EMP). Natural and synthetic mineral standards were used for calibration. Operating conditions were 15 kV accelerating voltage, 5 nA electron beam current and a spot size of 5 μm. Counting times were 5 s on the peak and 2 s on the background. Analytical data are summarized in online Supplementary Material Table S1.
For preparation of mineral separates for Rb–Sr analysis, hand specimens were cut into ∼10–20 mm thick rock slabs. Weathered crusts were removed, and fresh material was first crushed in a steel mortar and then its grain size was further reduced by repeated short grinding intervals in a tungsten carbide shatterbox. Following sieving, minerals were either enriched by adherence to a piece of paper or with a Frantz magnetic separator, followed by handpicking under the stereo microscope. Before dissolution, mineral concentrates were repeatedly rinsed in deionized H2O and in ethanol. Mineral separates (phengite: 2–12 mg; mostly <8 mg; epidote: 1–2 mg) and whole-rock powders (∼100 mg) were mixed with 87Rb–84Sr spike in Teflon vials and dissolved in HF–HNO3 (5:1) on a hot plate overnight. After complete evaporation, 6 N HCl was added to the residue. This mixture was again homogenized on a hot plate overnight. After a second evaporation to dryness, Rb and Sr were separated by standard ion exchange procedures (AG 50W-X8 resin) on quartz glass columns using 2.5 N HCl as the eluent.
For mass-spectrometric analysis, Sr was loaded with TaF5 on W filaments and measured in static mode using a Thermo Finnigan Triton thermal ionization mass spectrometer (TIMS). During the two analytical sessions of this study, the NIST SRM 987 reference material yielded 87Sr/86Sr values of 0.710292 ± 0.000015 (2σ; n = 10) and 0.710301 ± 0.000012 (2σ; n = 9), respectively. The 87Sr/86Sr values of samples are reported relative to 0.710248 for the SRM 987. Correction for mass fractionation is based on 86Sr/88Sr = 0.1194 using the exponential law. Data were corrected for total procedural blanks of 10 pg for Rb and 20 pg for Sr.
Rubidium concentrations were determined by isotope dilution with a multiple-collector inductively coupled plasma mass spectrometer (MC-ICP-MS) (Thermo Neptune Plus), using admixed Zr to correct for the instrumental mass bias (Nebel et al. Reference Nebel, Mezger, Scherer and Münker2005). The external reproducibility on the Rb/Sr of samples was estimated from the repeated analysis of optimally spiked standard solutions and samples, i.e. 0.1–0.2 %. This uncertainty was then multiplied by an error magnification factor to account for over- or underspiking (e.g. Stracke et al. Reference Stracke, Scherer, Reynolds, Holland and Turekian2014). For isochron calculations, 87Sr/86Sr values were assigned external 2σ uncertainties of 0.005 %. Data evaluation, age calculation and presentation in diagrams is based on Isoplot/Ex 4.15 (Ludwig, Reference Ludwig2012) using the 87Rb decay constant of Villa et al. (Reference Villa, De Bièvre, Holden and Renne2015). Isochron dates are reported with Model 1 solutions at the 95 % confidence level.
4. Samples
Five samples were selected for Rb–Sr geochronology. Sampling locations are illustrated in Figure 2, and GPS coordinates are reported in Table 1. Field images are shown in Figure 3. Photomicrographs are depicted in Figure 4 and online Supplementary Material Figure S1.
Table 1. Rb–Sr isotope data of blueschist-facies rocks from the Makrotantalon Unit

* The external reproducibility (2σ) of 87Rb/86Sr was estimated from the repeated analysis of optimally spiked standard solutions and samples, and includes an error magnification for under- or overspiking. The 2σm uncertainties on 87Sr/86Sr represent the internal analysis statistics (2 standard errors). For age calculations, all 87Sr/86Sr values were assigned an uncertainty of 0.005 % (2σ).
† Whole-rock powders were prepared from different grain-size fractions, not from a full sample.

Fig. 3. Field images from the Makrotantalon Unit. (a) Overview of the outcrop at Mikri Peza; BS – blueschist; GS – greenschist. (b) Close-up of epidote-glaucophane schist from this outcrop locality, indicated in (a) with arrow ‘BS’. (c, d) Greenschist-facies rock sequences forming most parts of the peninsula shown in (a) as exposed in the next bay (Meghali Peza). Hammer for scale in (b) and (d) is 30 cm in length.

Fig. 4. Thin-section photomicrographs of blueschist-facies samples from the Makrotantalon Unit that were used for Rb–Sr geochronology (plane-polarized light). (a) Sample 5776. (b) Sample 8104. (c) Sample 8111. (d) Sample 10AD37. (e) Sample 8192B. (f) Sample 1453, dated by Bröcker & Franz (Reference Bröcker and Franz2006) and originally assigned to the Lower Unit. Ph – phengite; Ep – epidote; Gln – glaucophane; Qz – quartz; Grt – garnet. Scale bar is 100 µm across.
Samples 5776 and 8104 (Fig. 4a, b) are strongly foliated and partially folded garnet-glaucophane schists from the Fellos area that were collected from the same lithological horizon in close vicinity to each other. The mineral assemblage mainly consists of garnet, glaucophane, phengite, quartz and rutile. Zircon is a typical accessory phase. Garnet occurs as porphyroclasts and is often strongly fragmented. Iron oxide-stained garnet is common. Heterogeneously distributed white mica and sodic amphibole define the foliation. Glaucophane is generally well-preserved and mostly lacks obvious signs of retrogression. Titanite, chlorite and a brown phyllosilicate (biotite or oxychlorite) document variable degrees of lower pressure overprinting.
Sample 8111 is a weakly crenulated epidote-glaucophane schist that was collected near the lighthouse at Fasa (Figs 2, 4c). This rock mainly consists of epidote, glaucophane and phengite. Quartz, titanite, apatite, tourmaline and opaque phases occur in minor or accessory modal quantities. Titanite was stable at peak conditions. Chlorite and albite are secondary.
Samples 10AD37 and 8192B are epidote-glaucophane schists from the area NE of Kalivari (Figs 2, 4d, e). The mineral assemblages are similar to that of sample 8111, but the rocks are more crenulated. In sample 10AD37, glaucophane has been partially replaced by a more calcic amphibole.
For details of the structural inventory and deformation history of NW Andros see Gerogiannis et al. (Reference Gerogiannis, Xypolias, Chatzaras, Aravadinou and Papapavlou2019).
5. Results
5.a. Mineral chemistry
To characterize the white mica populations and to identify mixed populations, polished thin-sections were prepared from splits of the phengite separates that were used for white mica isotopic analyses, with the basal plane of mica plates positioned parallel to the surface of the glass slide. This orientation allowed systematic and representative EMP analysis of core and near rim compositions, and for each sample, phengite from different sieve fractions was analysed (∼25–30 core–rim pairs each; online Supplementary Material Table S1). White mica compositions were also determined in situ in polished thin-sections. The Si-content was used as a proxy to evaluate inter- and intragrain compositional variability and inferred relative pressure differences (Massonne & Schreyer, Reference Massonne and Schreyer1987). Results are shown in Figures 5 and 6.

Fig. 5. Si versus Al diagrams (atoms per formula unit) for white mica of Makrotantalon samples representing different grain-size fractions of separated (isolated) grains and in situ analyses. The lines indicate a compositional trend from muscovite to aluminoceladonite.

Fig. 6. White mica compositions (atoms per formula unit) of Makrotantalon samples. (a) Si rim versus Si core; 1:1 reference line indicates no zoning. (b) Si versus XNa diagram.
Most white mica populations are paragonite-free and only consist of phengite. Small amounts of paragonite were only recognized in sample 8104. Separated grains are characterized by rather homogeneous compositions, and no difference between different grain-size fractions is evident (Fig. 5; online Supplementary Material Table S1). Most of these grains show only slight zoning of Si-values without a uniform trend (Fig. 6a).
Two generations of phengite were recognized in the in situ data of samples 5776 and 8104, which comprise crystals with Si-values of 3.43–3.55 and 3.22–3.34, respectively, and also differ in their XNa-values (<0.1 and 0.11–0.20; Figs 5a, d, 6b; online Supplementary Material Table S1). The dataset of separated grains from these samples almost completely comprises phengite of the low-Si group (3.23–3.33), which is characterized by elevated Na2O concentrations (0.8–1.6 wt %) and lower K2O contents (8.8–10.8 wt %) compared to the high-Si phengites. The XNa (Na/(Na + K + Ca)) value is typically 0.11–0.21, and XMg (Mg/(Mg + Fe + Mn)) values are in the 0.63–0.73 range.
White mica of samples 8111 and 8192B show little intra- and inter-sample variations and are characterized by Si-values of 3.37–3.55 and 3.38–3.59, respectively, without compositional differences between isolated and in situ analyses (Fig. 5g–l). The XMg value is in the range from 0.40 to 0.69 and XNa is <0.1. In situ analysed phengite of sample 10AD37 has similar compositional characteristics (Si: 3.44–3.57; XMg: 0.48–0.71; XNa: <0.1).
Most amphiboles are glaucophane, but the compositional range extends slightly into the ferro-glaucophane and magnesio-riebeckite fields (Fig. 7). Two compositional groups can be distinguished. Sodic amphiboles of samples 5776 and 8104 are characterized by XMg (Mg/(Mg + Fe2+)) of 0.47–0.57 and XFe3+ ((Fe3+/(Fe3+ + AlVI)) of 0.07–0.17. In the other samples, XMg and XFe3+ are 0.59–0.86 and 0.29–0.6, respectively. Epidotes have pistacite values (XFe3+ = Fe3+/(Fe3+ + Al)) of 0.25–0.31. The concentrations of Cr2O3 reach up to 0.33 wt % but are mostly <0.1 wt %. The MnO contents are in the range of 0.16–0.79 wt %.

Fig. 7. Amphibole classification diagrams (atoms per formula unit, based on Locock, Reference Locock2014) of Makrotantalon samples. (a) Mg/(Mg + Fe2+) versus Fe3+/(Fe3+ + VIAl) diagram. (b) Mg/(Mg + Fe2+) versus Si (Leake et al. Reference Leake, Woolley, Arps, Birch, Gilbert, Grice, Hawthorne, Kato, Kisch, Krivovichev, Linthout, Laird, Mandarino, Maresch, Nickel, Rock, Schumacher, Smith, Stephenson, Ungaretti, Whittaker and Youzhi1997). C – core; R – rim.
5.b. Rb–Sr geochronology
The Rb–Sr isochrons are based on different size fractions obtained by sieving of crushed rock. Coarser sieve fractions probably contain mostly grains that were originally coarse. Fine sieve fractions probably contain a mix of originally fine grains and broken fragments of originally coarser grains. In the case of fully equilibrated rocks, this will not affect the isochron age. However, complexities can arise from inheritance and disequilibria, which can often be identified by the systematic deviation of the smallest or largest sieve size fractions from the regression line.
Analytical data are summarized in Table 1 and shown in Figure 8. Phengite grains of samples 5776 and 8104 have relatively high Sr concentrations of ∼120–170 ppm, whereas white mica of the other samples is characterized by Sr contents of <10–28 ppm. Epidote splits of sample 8111 have relatively high Rb concentrations (15–18 ppm) indicating imperfect purification or the presence of white mica inclusions. This has no significant effect on the isochron plots because of the high Sr concentrations of the epidotes (1400–1500 ppm).
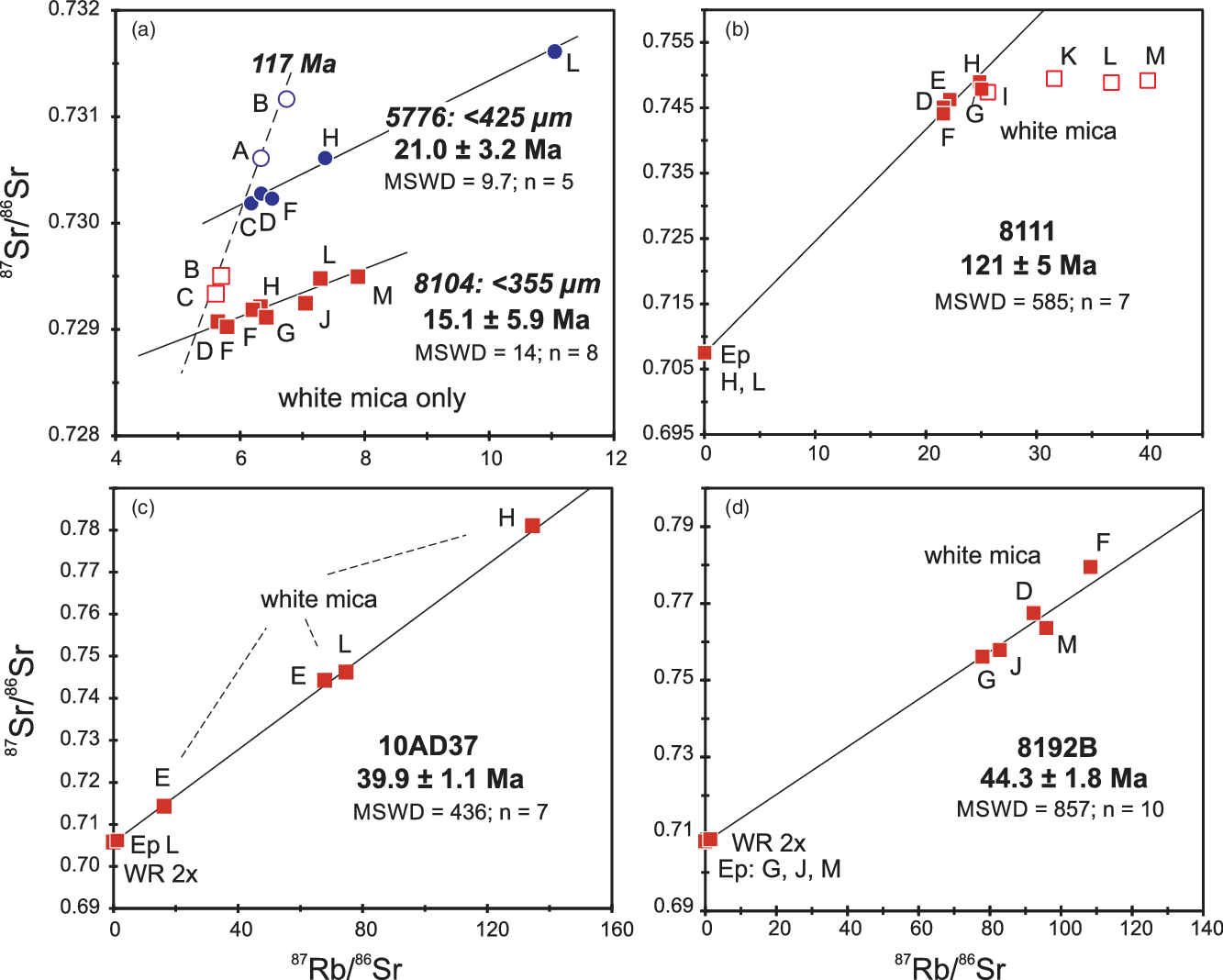
Fig. 8. Rb–Sr isochron diagrams for blueschist-facies samples from the Makrotantalon Unit. Ph – phengite; Ep – epidote. Uncertainties are smaller than symbol size. A, B, C, etc. refer to sieved grain-size fractions indicated in Table 1.
Apparent ages of the garnet-glaucophane schists 5776 and 8104 are based on a set of different sieve fractions of phengite. In both cases, linear regression indicates strong scatter. For sample 5776, the <425 µm grain-size fractions indicate an apparent age of 21.0 ± 3.2 Ma (Mean Square Weighted Deviation, MSWD = 9.7; Fig. 8a). Eight <355 µm grain-size fractions of sample 8104 mica yielded a Rb–Sr date of 15.1 ± 5.9 Ma (MSWD = 14; Fig. 8a). The excluded two larger grain-size fractions of samples 5776 and 8104 plot along a 117 Ma reference line (Fig. 8a). For sample 8111, 11 data points were determined, representing different sieve fractions of phengite (9x) and epidote (2x). Excluding the <200 µm phengite splits results in a straight-line fit with a Rb–Sr date of 121 ± 5 Ma (MSWD = 585; Fig. 8b). White mica (4x) and epidote (1x) aliquots of sample 10AD37 yielded a Rb–Sr date of 39.9 ± 2.0 Ma (MSWD = 727), and of 39.9 ± 1.1 Ma (MSWD = 436; Fig. 8c), if two whole-rock analyses are included. In the case of sample 8192B, alignment of data points representing sized fractions of phengite (5x) and epidote (3x) yielded a Rb–Sr date of 44.3 ± 2.2 Ma (MSWD = 1103). Inclusion of the whole-rock data points into the linear regression gives an apparent age of 44.3 ± 1.8 Ma (MSWD = 857; Fig. 8d).
6. Discussion
Previous studies indicated that the Makrotantalon Unit records a complex polymetamorphic history, including an Early Cretaceous blueschist-facies event, a Late Cretaceous greenschist- to amphibolite-facies episode, an Eocene HP/LT metamorphic event and a Miocene greenschist-facies overprint (Bröcker & Franz Reference Bröcker and Franz2006; Huyskens & Bröcker, Reference Huyskens and Bröcker2014; Huet et al. Reference Huet, Labrousse, Monié, Malvoisin and Jolivet2015; Gerogiannis et al. Reference Gerogiannis, Xypolias, Chatzaras, Aravadinou and Papapavlou2019). In the following, we critically evaluate interpretations suggesting that the Makrotantalon Unit was affected by two blueschist-facies events at different times (Gerogiannis et al. Reference Gerogiannis, Xypolias, Chatzaras, Aravadinou and Papapavlou2019) using new and existing geochronological data.
Peak metamorphic temperatures of the Makrotantalon blueschists (∼550 °C; Huet et al. Reference Huet, Labrousse, Monié, Malvoisin and Jolivet2015) are close to the commonly cited empirical bulk closure temperature for the Rb–Sr isotope system of white mica (∼500–550 °C; e.g. Purdy & Jäger, Reference Purdy and Jäger1976; von Blanckenburg et al. Reference von Blanckenburg, Villa, Baur, Morteani and Steiger1989). This suggests that the new mineral isochron dates were not significantly affected by cooling, but instead indicate the time of (re)crystallization or subsequent disturbance by infiltrating fluids. The similarity with the Ar–Ar white mica dates (closure temperature ∼400–440 °C, e.g. Harrison et al. Reference Harrison, Celerier, Aikman, Hermann and Heizler2009; Scharf et al. Reference Scharf, Handy, Schmid, Favaro, Sudo, Schuster and Hammerschmidt2016) indicates fast exhumation and is consistent with this interpretation.
The Rb–Sr regressions yielded high MSWD values for all studied samples. This value provides a goodness-of-fit parameter for data points plotting along the regression line, which defines the age-dependent slope of the isochron. In the present case, these values cannot be further optimized by rejection of individual data points from the regression. This would only be justified in the case of obvious disequilibrium. In general, the MSWD is controlled by a combination of geological factors and analytical precision. The scatter of data points beyond analytical error (leading to large MSWD values) is a common issue affecting incompletely recrystallized polymetamorphic or polydeformed samples (e.g. Halama et al. Reference Halama, Glodny, Konrad-Schmolke and Sudo2018). Furthermore, for a given amount of real geologic scatter in Andros Rb–Sr regressions, the MSWD will be higher when the analytical uncertainties are smaller. In TIMS studies, the 87Rb/86Sr values are often reported with 1–1.5 % uncertainty, which is estimated from replicate analyses of unspiked or spiked samples or reference materials. In contrast, MC-ICP-MS allows a more precise determination of the Rb isotope ratio by correcting the instrumental mass bias using admixed Zr, leading to a much better reproducibility of the measurements (e.g. Nebel et al. Reference Nebel, Mezger, Scherer and Münker2005). For example, the uncertainty of the 87Rb/86Sr phengite data (MC-ICP-MS) of samples 8111, 10AD37 and 8192B is mostly <0.4 %, resulting in MSWD values of 585, 436 and 857, respectively. In contrast, a more TIMS-typical uncertainty of 1 % on the 87Rb/86Sr ratios would result in MSWD values of 36, 41 and 89, respectively. These values are still indicative of errorchrons (e.g. Wendt & Carl, Reference Wendt and Carl1991), but a high MSWD is not a priori an indication of geological irrelevance (e.g. Kalsbeek & Hansen, Reference Kalsbeek and Hansen1989; Halama et al. Reference Halama, Glodny, Konrad-Schmolke and Sudo2018). Since the apparent ages coincide with existing Ar–Ar and Rb–Sr dates for similar rocks from the study area, the new results are considered geologically meaningful, closely approximating the true metamorphic ages.
6.a. Cretaceous HP/LT metamorphism in the Makrotantalon Unit?
In the Fellos area (Fig. 2), HP/LT rocks are exposed below a serpentinite body that extends over several hundred metres along a mountain ridge (Mukhin, Reference Mukhin1996). Huyskens & Bröcker (Reference Huyskens and Bröcker2014) interpreted the serpentinite as a lithological marker of the tectonic contact between the Makrotantalon and the Lower Unit, whereas Xypolias et al. (Reference Xypolias, Gerogiannis, Chatzaras, Papapavlou, Kruckenberg, Aravadinou and Michels2018) argued that the contact is located a few hundred metres beneath the serpentinite. An epidote-glaucophane schist collected in the lower part of the metasedimentary succession below the serpentinite body yielded an Eocene Rb–Sr date (45.7 ± 3.2 Ma; Bröcker & Franz, Reference Bröcker and Franz2006). At a similar lithostratigraphic position elsewhere on the island, relics of Na-amphibole are also sporadically preserved in strongly overprinted rocks. Most of these greenschist-facies samples yielded white mica Rb–Sr dates of ∼40 Ma (Huyskens & Bröcker, Reference Huyskens and Bröcker2014). The Eocene dates were interpreted as a legacy of the HP/LT event recorded in the Cycladic Blueschist Unit, and the sampling locations were therefore considered to belong to the Lower Unit (Huyskens & Bröcker, Reference Huyskens and Bröcker2014).
Huyskens & Bröcker (Reference Huyskens and Bröcker2014) reported clear evidence for blueschist-facies metamorphism from other locations in the Makrotantalon Unit but did not find suitable glaucophane-bearing samples for white mica geochronology that could unambiguously be assigned to this nappe. These authors assumed that both the Makrotantalon Unit and the Lower Unit were affected by a single blueschist-facies event in the Eocene and concluded that the Makrotantalon Unit is a distinct tectonic subunit of the Cycladic Blueschist Unit. This interpretation was challenged by 40Ar–39Ar single phengite dating of a glaucophane-garnet schist from a higher lithostratigraphic position in the Fellos succession that yielded Cretaceous dates (117.2 ± 1.8 Ma, 115.6 ± 1.9 Ma, 105.9 ± 1.7 Ma; Huet et al. Reference Huet, Labrousse, Monié, Malvoisin and Jolivet2015). The youngest date overlaps with apparent Rb–Sr ages of greenschist-facies rocks (104.6 ± 3.8 Ma and 103.9 ± 1.3 Ma; Bröcker & Franz, Reference Bröcker and Franz2006), which was interpreted as an indication that a contamination with excess Ar is insignificant and that the 40Ar–39Ar dates represent geologically meaningful crystallization ages. However, this argument is not fully convincing because the 40Ar–39Ar and Rb–Sr dates were determined on rocks having different metamorphic histories.
In the Fellos area, garnet-glaucophane schists occur within a sequence of siliciclastic metasedimentary rocks. Huet et al. (Reference Huet, Labrousse, Monié, Malvoisin and Jolivet2015) reported that the Ar–Ar-dated sample was collected within the serpentinites. Our field observations indicate that such rocks only occur below the serpentinite, but judging from their sample description, the Rb–Sr dated samples 5776 and 8104 correspond to the same rock type that yielded the Cretaceous 40Ar–39Ar dates.
White mica geochronology of rocks with a complex, multi-phase tectonometamorphic evolution is often compromised by incomplete resetting of the isotopic system or the presence of different mica generations (e.g. Bröcker et al. Reference Bröcker, Baldwin and Arkudas2013; Kurzawa et al. Reference Kurzawa, Bröcker, Fotoohi Rad, Berndt and Lisker2017). The phengite population of the Ar–Ar-dated sample included two compositional groups with Si-values of ∼3.4 and ∼3.2, respectively, that were recognized both in situ and in isolated single grains left over from dating by laserprobe step heating (Huet et al. Reference Huet, Labrousse, Monié, Malvoisin and Jolivet2015). These authors concluded that the high-Si phengite crystallized during the HP/LT stage, whereas white mica with lower Si-values formed together with chloritized biotite during later retrogression. Two of the Ar–Ar-dated phengite grains had Si-rich cores and Si-poor rims (fig. 3 in Huet et al. Reference Huet, Labrousse, Monié, Malvoisin and Jolivet2015). Judging from the coincidence of the Ar–Ar plateau and total fusion ages (Huet et al. Reference Huet, Labrousse, Monié, Malvoisin and Jolivet2015), the volume proportion of the low-Si rims was small and had a negligible influence on the overall age.
For the newly dated samples, we determined the phengite chemistry of separated grains from the same mineral concentrates that were used for preparation of aliquots for Rb–Sr isotope analyses, according to the same screening criteria. In the case of the Fellos samples 5776 and 8104, no significant compositional differences were recognized between individual grain-size fractions, and many of the Si-values cluster around 3.3–3.2 (Fig. 5). On the other hand, EMP in situ measurements of randomly selected spots clearly indicate the existence of a bimodal phengite population (Fig. 5a, d). Since the micas of these samples were only enriched by adherence to a piece of paper and not by magnetic properties, a selective pre-concentration during earlier mineral separation steps can be ruled out. In both samples, the mica grains are often very rich in opaque phases. A plausible explanation for the lack of the high-Si micas in the group of separated grains is that such grains were removed during handpicking under the binocular microscope because of abundant inclusions or signs of alteration.
Owing to disturbance of the Rb–Sr isotope system, samples 5776 and 8104 only yielded errorchrons (Fig. 8a), which document a combination of incomplete resetting and partial recrystallization. The larger phengite grain-size fractions do not fit on a common regression line with the smaller grain-size fractions. The apparent lack of a correlation between the Si-values of the various mica sieve fractions and their deviation from a common Rb–Sr regression line suggests that readjustment of the major elements and isotopic characteristics are not correlated or that mixing of the mica populations is not fully reflected by the available EMP data.
The coarser (>355 µm) phengite fractions of samples 5776 (A & B) and 8104 (B & C) lie on a trend indicating a Rb–Sr age of c. 117 Ma (Fig. 8a). As these samples were collected close to each other from the same lithologic horizon, it can be reasonably assumed that they had the same or a very similar initial 87Sr/86Sr value. The smaller grain-size fractions alone define trends consistent with various Miocene dates (Fig. 8a). In the case of sample 5776, the apparent age of phengite grains <425 µm (sieve fractions C–L) is c. 21 Ma (Fig. 8a; Table 1). A subset of sample 8104 phengite analyses (<355 µm, sieve fractions D–M) yielded an errorchron date of 15 ± 6 Ma (Fig. 8a; Table 1).
Scatter observed for replicate analyses of the same or a similar grain-size range indicate that the original (in situ) grain-size distribution has not been completely preserved by sieving. Individual sieve fractions comprise different proportions of whole grains of the given sieve size and fragments of larger grains. However, this mixing effect is only of limited importance because handpicking has apparently removed the older high-Si phengites. The smaller phengite sieve fractions mainly consist of grains recording younger, deformation-related apparent ages with some fragments of coarser-grained, low-Si phengite that had already undergone partial resetting. As such, different sieve size fractions record different degrees of partial re-equilibration because of mixing during the crushing process, but these fractions are dominated by more strongly overprinted phengite.
We speculate that the Miocene dates are not a fortuitous result but most likely represent different increments of mica recrystallization during post-HP metamorphic deformation. There is a clear trend towards younger apparent ages for the smaller phengite grain-size fractions suggesting deformation-related resetting and synkinematic recrystallization of an older, presumably Cretaceous mica population. The Miocene dates cannot be linked with blueschist-facies metamorphism, but they do correspond very well to apparent ages for greenschist-facies overprinting and related tectonic processes in the nappe stack of the Cycladic Blueschist Unit (e.g. Ring et al. Reference Ring, Glodny, Will and Thomson2010; Bröcker et al. Reference Bröcker, Baldwin and Arkudas2013; Glodny & Ring, Reference Glodny and Ring2022 and references therein). The Cretaceous 40Ar–39Ar dates from the Fellos area could not be reproduced by Rb–Sr dating, but this is not indicative for contamination by extraneous Ar, but rather results from dating of different mica generations. For 40Ar–39Ar laserprobe step heating, Huet et al. (Reference Huet, Labrousse, Monié, Malvoisin and Jolivet2015) used phengite grains of the 0.5–1 mm size fraction. These larger grains are more likely to retain their original Si-values and age than the size fractions used for Rb–Sr dating but are poorly represented in the newly dated samples. We speculate that 40Ar–39Ar dating of smaller phengite grain sizes would also provide post-Cretaceous dates and that Rb–Sr dating on larger grain-size fractions than measured here would return Cretaceous ages.
Huet et al. (Reference Huet, Labrousse, Monié, Malvoisin and Jolivet2015) suggested that the different Ar–Ar dates (c. 116 and c. 106 Ma) correspond to peak metamorphic conditions and a later stage on the retrogression path, respectively, but the presumed link between white mica composition and Ar–Ar dates could not be documented. Although we largely agree with this interpretation, the geological significance of the youngest Ar–Ar date remains ambiguous as the K–Ar system could have been disturbed at various stages during exhumation. We consider a relationship to Miocene deformation as a reasonable possibility.
In the Fellos area, intense localized ductile shear led to partial recrystallization of phengite in garnet-glaucophane schists at ∼50–100 m above the original tectonic contact. This process had almost no retrograde effect on associated glaucophane. The Miocene Rb–Sr dates are likely related to the formation of the Fellos shear zone (Xypolias et al. Reference Xypolias, Gerogiannis, Chatzaras, Papapavlou, Kruckenberg, Aravadinou and Michels2018), which overprinted and transposed the original nappe contact during exhumation. This is also supported by the fact that blueschist-facies D2 microstructures on both sides of the tectonic contact indicate that the tectonic juxtaposition of the Makrotantalon Unit with the Lower Unit occurred before or during earlier HP/LT metamorphism. The Fellos shear zone is a NE-striking, dextral transpressional zone that developed in the short limb of an inclined antiform and can be mapped along strike over at least 1.5 km with a thickness of ∼250 m (Xypolias et al. Reference Xypolias, Gerogiannis, Chatzaras, Papapavlou, Kruckenberg, Aravadinou and Michels2018). The Rb–Sr dated samples record strain localization below serpentinites that outline the upper boundary of the shear zone.
Although the picture turned out to be more complicated than expected, the geological relevance of Cretaceous HP/LT metamorphism in the Makrotantalon Unit is also confirmed by Rb–Sr geochronology. The epidote-glaucophane schist 8111, which was collected in a different part of the study area, yielded an apparent age of 121 ± 5 Ma (Fig. 8b). This sample also records disturbance of the Rb–Sr isotopic system, mainly affecting the <180 µm size fractions, but the Cretaceous age was preserved by the larger phengite grains.
6.b. Eocene HP/LT metamorphism in the Makrotantalon Unit?
The subdivision of the Cycladic nappe stack into distinct subunits is often problematic, and the geology of NW Andros illustrates this situation very well. Gerogiannis et al. (Reference Gerogiannis, Xypolias, Chatzaras, Aravadinou and Papapavlou2019) concluded that the Makrotantalon Unit is an integral part of the Cycladic Blueschist Unit because their field observations and structural analysis indicated that the sampling location of a blueschist-facies rock with an Eocene age (45.7 ± 3.2 Ma; Bröcker & Franz, Reference Bröcker and Franz2006) from the Fellos area, originally interpreted as part of the Lower Unit, is instead located in the Makrotantalon Unit. The map of Gerogiannis et al. (Reference Gerogiannis, Xypolias, Chatzaras, Aravadinou and Papapavlou2019) also indicated a new tectonic assignment for greenschist-facies samples with similar Rb–Sr dates (45−38 Ma) that were also previously interpreted to belong to the Lower Unit (Huyskens & Bröcker, Reference Huyskens and Bröcker2014). Combined with the lack of HP/LT evidence in the Upper Cycladic Unit, the revised structural position of samples with Eocene ages was a key argument for linking the Makrotantalon Unit with the nappe stack of the Cycladic Blueschist Unit, and the interpretation that both share a common post-Cretaceous tectonometamorphic history (Gerogiannis et al. Reference Gerogiannis, Xypolias, Chatzaras, Aravadinou and Papapavlou2019). Despite considerable progress in understanding of the contact relationships, the exact position of the tectonic contact at the base of the Makrotantalon Unit remains ambiguous. Trace-element data from ultramafic rocks collected on both sides of the newly defined tectonic contact reveal almost identical geochemical characteristics, possibly indicating the existence of a wider fault zone (Höhn et al. Reference Höhn, Bröcker and Berndt2022). Structural and mineralogical similarities on both sides of the tectonic contact and the lobate pattern for the fault zone, interpreted to result from complex exhumation-related folding (Gerogiannis et al. Reference Gerogiannis, Xypolias, Chatzaras, Aravadinou and Papapavlou2019), may have locally obscured identification of the exact position of the tectonic contact and thus the correct structural assignment of samples collected near the inferred nappe boundary. However, the interpretation that the Makrotantalon Unit records Eocene HP/LT metamorphism is supported by Rb–Sr dates (39.9 ± 1.1 Ma and 44.3 ± 1.8 Ma; Fig. 8c, d) for two newly dated epidote-glaucophane schists (samples 10AD37 and 8192B), which were collected at a greater distance from the tectonic contact than the sampling location of the Fellos area (Bröcker & Franz, Reference Bröcker and Franz2006). Despite the high MSWD values, the most straightforward explanation for the trends shown (Fig. 8c, d) is a basically isochronous relationship, where either full initial isotopic equilibration was not achieved, or a later disturbance occurred without erasing the original age. Both newly dated samples were affected by a greenschist-facies overprint as indicated by the presence of secondary chlorite and albite. There is no obvious link to retrograde deformation and thus the scatter in the isochron diagrams (Fig. 8e, f) is ascribed to disturbance of the Rb–Sr isotope system during fluid-assisted overprinting at lower pressure.
Bröcker et al. (Reference Bröcker, Huyskens and Berndt2016) reported U–Pb zircon ages of siliciclastic rocks from Andros which indicated maximum depositional ages of ∼260 Ma for the Makrotantalon Unit and of 150–125 Ma for the Lower Unit. According to more recent findings, however, the sampling location of the garnet-glaucophane schist 5775, originally interpreted to represent the topmost part of the Lower Unit (Bröcker et al. Reference Bröcker, Huyskens and Berndt2016), corresponds instead to the lowermost part of the Makrotantalon Unit (Huet et al. Reference Huet, Labrousse, Monié, Malvoisin and Jolivet2015; Xypolias et al. Reference Xypolias, Gerogiannis, Chatzaras, Papapavlou, Kruckenberg, Aravadinou and Michels2018; Gerogiannis et al. Reference Gerogiannis, Xypolias, Chatzaras, Aravadinou and Papapavlou2019). This sample was collected close to the Rb–Sr dated samples 5776 and 8104 and represents the same rock type that yielded the Cretaceous 40Ar–39Ar dates (Huet et al. Reference Huet, Labrousse, Monié, Malvoisin and Jolivet2015). The youngest zircon rim age (43.9 ± 4.8 Ma; 2σ) of the detrital population closely corresponds to the Rb–Sr date of the HP/LT schist (45.7 ± 3.2 Ma; Bröcker & Franz, Reference Bröcker and Franz2006) collected nearby, and clearly documents the influence of Eocene zircon-forming processes on rocks recording Cretaceous blueschist-facies metamorphism. A relationship to the Eocene HP/LT event is a plausible interpretation.
6.c. Is the Makrotantalon Unit an integral part of the Cycladic Blueschist Unit?
Early Cretaceous ages (∼116 Ma) of blueschist-facies rocks clearly distinguish the Makrotantalon Unit from other subunits of the Cycladic Blueschist Unit, which lack conclusive evidence for a pre-Eocene metamorphic history. Together with Late Cretaceous dates (∼100–90 Ma and ∼80–70 Ma) for greenschist-facies rocks from the same tectonic unit, these ages suggest a correlative relationship to the Pelagonian Zone on the Greek mainland (Bröcker & Franz, Reference Bröcker and Franz2006; Huet et al. Reference Huet, Labrousse, Monié, Malvoisin and Jolivet2015). The geological relevance of Cretaceous 40Ar–39Ar dates of glaucophanes from south Evia (∼120–110 Ma; Maluski et al. Reference Maluski, Vergely, Bavay, Bavay and Katsikatsos1981) has not been corroborated yet, but these apparent ages broadly correspond to the Cretaceous white mica dates of the Makrotantalon Unit (Huet et al. Reference Huet, Labrousse, Monié, Malvoisin and Jolivet2015; this study), suggesting geological significance and a similar correlative relationship.
Huet et al. (Reference Huet, Labrousse, Monié, Malvoisin and Jolivet2015) assumed that the metamorphic evolution of the Makrotantalon Unit includes a Cretaceous HP/LT event but not an Eocene one, and that both the Makrotantalon Unit and the structurally lower Cycladic Blueschist Unit were affected by the same lower pressure history. Following the hypothesis of tectonic emplacement at ∼40 Ma (Huyskens & Bröcker Reference Huyskens and Bröcker2014), Huet et al. (Reference Huet, Labrousse, Monié, Malvoisin and Jolivet2015) assumed that the Cycladic Blueschist Unit was affected by blueschist-facies metamorphism when the Pelagonian-derived Makrotantalon Unit was still a part of the upper plate of the subduction system. This led Huet et al. (Reference Huet, Labrousse, Monié, Malvoisin and Jolivet2015) to the conclusion that the tectonic contact at the base of the Makrotantalon Unit was a normal fault and not a thrust (Papanikolaou, Reference Papanikolaou1978 b; Huyskens & Bröcker, Reference Huyskens and Bröcker2014). However, confirmation of additional Eocene HP/LT metamorphism is at variance to a model that postulates a normal fault at the base of the Makrotantalon Unit.
The existence of Eocene blueschists is a key argument for the interpretation that the Makrotantalon Unit is an integral part of the Cycladic Blueschist Unit (Gerogiannis et al. Reference Gerogiannis, Xypolias, Chatzaras, Aravadinou and Papapavlou2019). However, a relationship to the nappe stack of the Cycladic Blueschist Unit cannot unambiguously be deduced from geochronological data alone, because Eocene HP/LT rocks have also been described from the Pelagonian Zone (Schermer et al. Reference Schermer, Lux and Burchfiel1990; Lips et al. Reference Lips, White and Wijbrans1998, Reference Lips, Wijbrans, White, Durand, Jolivet, Horvàth and Séranne1999).
The NW-trending Pelagonian Zone represents the westernmost part of the Internal Hellenides and includes rock sequences that have undergone Cretaceous to Eocene blueschist- and greenschist-facies metamorphism (e.g. Schermer, Reference Schermer1990; Schermer et al. Reference Schermer, Lux and Burchfiel1990; Lips et al. Reference Lips, White and Wijbrans1998, Reference Lips, Wijbrans, White, Durand, Jolivet, Horvàth and Séranne1999). The main occurrences of such rocks are the Mt Olympos and Ossa tectonic windows (Fig. 1a) where a weakly metamorphosed sequence of autochthonous platform carbonates and phyllitic rocks is overlain by an allochthonous basement with Hercynian granites and gneisses. In contrast to the HP/LT rocks of the Cyclades (including the Makrotantalon Unit), which have reached P–T conditions of the eclogite- to epidote-blueschist-facies (e.g. Huet et al. Reference Huet, Labrousse, Monié, Malvoisin and Jolivet2015; Laurent et al. Reference Laurent, Lanari, Naïr, Augier, Lahfid and Jolivet2018), lower grade metamorphic conditions were estimated for the Pelagonian blueschist-greenschist and blueschist-facies rocks (Mt Olympos: <350–500 °C and 0.5–0.8 GPa, Schermer et al. Reference Schermer, Lux and Burchfiel1990; Ossa: 350 °C and 0.8 GPa, Lips et al. Reference Lips, White and Wijbrans1998). Schermer et al. (Reference Schermer, Lux and Burchfiel1990) reported multigrain 40Ar–39Ar and Rb–Sr isochron dates indicating four major deformational and metamorphic events at ∼293 Ma, ∼100 Ma, 61–53 Ma and 40–36 Ma. The oldest ages represent crystallization and cooling of the granitic basement sequence. Apparent ages of ∼100 Ma in the autochthonous sequences were interpreted as time constraints of greenschist- to blueschist-facies metamorphism and contemporaneous tectonic stacking of thrust sheets. The age groups at 61–53 Ma and 40–36 Ma were related to blueschist-facies metamorphism and thrusting of blueschists over a carbonate platform, respectively (Schermer et al. Reference Schermer, Lux and Burchfiel1990). Still younger ages between 23 Ma and 16 Ma were linked to uplift and cooling below 150 °C. On the basis of single-grain 40Ar–39Ar laserprobe dating, Lips et al. (Reference Lips, White and Wijbrans1998, Reference Lips, Wijbrans, White, Durand, Jolivet, Horvàth and Séranne1999) concluded that HP/LT metamorphism in the Ossa tectonic window and the southern Pelion Massif began at ∼100–85 Ma and ceased at ∼54 Ma, followed by greenschist-facies metamorphism that ended in the Ossa–Olympos region by 40–36 Ma but continued in the Pelion Massif until 15 Ma.
Similarities in the post-Cretaceous geochronological record of the Pelagonian Zone and the Cycladic Blueschist Unit alone do not provide an obvious argument for an unambiguous assignment of the Makrotantalon Unit to the nappe stack of the Cycladic Blueschist Unit. However, microstructural observations further support this interpretation. Gerogiannis et al. (Reference Gerogiannis, Xypolias, Chatzaras, Aravadinou and Papapavlou2019) related the main ductile deformation phase affecting both tectonic units exposed in NW Andros to tectonic stacking. A well-preserved penetrative to mylonitic blueschist-facies foliation on both sides of the tectonic contact was linked to the Eocene HP/LT event that is recorded on a regional scale in the Cyclades. An earlier deformation phase at blueschist-facies conditions is only preserved within the Makrotantalon Unit and is possibly associated with the Cretaceous HP/LT event (Gerogiannis et al. Reference Gerogiannis, Xypolias, Chatzaras, Aravadinou and Papapavlou2019). Combined, these observations further substantiate the interpretation that the Miocene dates of the Fellos samples are related to tectonic transposition of the contact between the two nappes during exhumation. Despite its Pelagonian origin (Huet et al. Reference Huet, Labrousse, Monié, Malvoisin and Jolivet2015), the Makrotantalon Unit is now an integral part of the Cycladic Blueschist Unit with a common metamorphic history from Eocene time onwards (Gerogiannis et al. Reference Gerogiannis, Xypolias, Chatzaras, Aravadinou and Papapavlou2019). The inferred sequence of events that led to the incorporation of the Pelagonian Makrotantalon Unit into the nappe stack of the Cycladic Blueschist Unit is schematically shown in Figure 9. This model follows Gerogiannis et al. (Reference Gerogiannis, Xypolias, Chatzaras, Aravadinou and Papapavlou2019), who suggested tectonic juxtaposition of these units at deep subduction levels during Eocene time or somewhat earlier, resulting in a common metamorphic history since that time.

Fig. 9. Proposed tectonic model for the study area from the Late Cretaceous to the Miocene showing the inferred sequence of events that led to incorporation of the Pelagonian Makrotantalon Unit into the nappe stack of the Cycladic Blueschist Unit and the subsequent exhumation (modified after Gerogiannis et al. Reference Gerogiannis, Xypolias, Chatzaras, Aravadinou and Papapavlou2019; see text for explanation).
7. Summary and conclusion
Using Rb–Sr geochronology, we dated blueschist-facies rocks from the Makrotantalon Unit on Andros, Greece. We assume that all samples were originally fully equilibrated on the whole-rock scale under HP/LT conditions at different times. The poor linear fit in the multi-point isochron diagrams and related high MSWD values indicate scatter in excess of analytical uncertainty, resulting from disturbance of the Rb–Sr isotope system during post-HP/LT metamorphic processes. This study is not providing high-precision geochronology, but rather shows that a disturbed dataset can still make sense in the regional chronological framework. The new apparent ages are consistent with existing Ar–Ar and Rb–Sr data of similar rocks from the study area and are considered to place geologically meaningful constraints on the time of two distinct blueschist-facies events that occurred c. 60–70 Ma apart.
The results of this study clearly show that the Makrotantalon Unit was affected by both Cretaceous and Eocene HP/LT episodes, and they substantiate interpretations suggesting that the Makrotantalon Unit represents a tectonic slice with Pelagonian affinity in the nappe stack of the Cycladic Blueschist Unit (Huet et al. Reference Huet, Labrousse, Monié, Malvoisin and Jolivet2015; Gerogiannis et al. Reference Gerogiannis, Xypolias, Chatzaras, Aravadinou and Papapavlou2019). The studied samples are affected by incomplete re-equilibration of the Rb–Sr isotope system and document a combination of inheritance from the HP/LT stage and partial recrystallization during lower pressure overprinting and deformation. The smaller grain-size fractions yielded Miocene Rb–Sr dates that are related to synkinematic recrystallization of micas during the formation of exhumation-related shear zones, which have transposed the original thrust contact. These dates are consistent with apparent ages of greenschist-facies rocks from the Lower Unit on Andros and elsewhere in the Cycladic Blueschist Unit. Epidote-glaucophane schists collected at a greater distance from the tectonic contact in other parts of the Makrotantalon Unit are also characterized by disturbed isochrons, but a relationship to tectonic stacking or later fault reactivation is not evident from observations and data. As glaucophane is partially replaced by retrograde phases, the scatter in the isochron plots can broadly be associated with greenschist-facies overprinting. The Rb–Sr dates of such samples (c. 121 Ma, c. 40 Ma and c. 44 Ma) further substantiate the significance of both Cretaceous and Eocene blueschist-facies events in the Makrotantalon Unit.
Supplementary material
To view supplementary material for this article, please visit https://doi.org/10.1017/S0016756822000280
Acknowledgements
This work was supported by a grant of the Deutsche Forschungsgemeinschaft (BR 1068/26-1). We thank Heidi Baier for supporting Rb–Sr analyses and Thomas Lamont for constructive comments on an earlier version of the manuscript.