1. Introduction
In the Keszthely Hills (KH) and the Southern Bakony Mountains (SBM), kaolin deposits fill ~100 m deep karstic sinkholes of a planation surface formed on Upper Triassic carbonate rocks. The study area is part of the Transdanubian Range (TR) that belongs to the Alpine–Carpathian–Pannonian (ALCAPA) composite terrain (Fig. 1; Balázs et al. Reference Balázs, Matenco, Magyar, Horváth and Cloetingh2016 and references therein). Several erosional events since the Albian resulted in large stratigraphic gaps in the carbonate-dominated Triassic to Miocene sequences and led to the formation of now fossilized palaeosinkholes and dolinas in the Upper Triassic carbonates (Csillag & Sebe, Reference Csillag, Sebe and Lóczy2015). The karstic depressions trapped strongly altered weathering products, such as kaolin, red clay and/or bauxite deposits, from the contemporaneously exposed basement and siliciclastic assemblages (Budai et al. Reference Budai, Császár, Csillag, Dudko, Koloszár, Majoros and Budai1999; Mindszenty et al. Reference Mindszenty, Csoma, Török, Hips and Hertelendi2000).
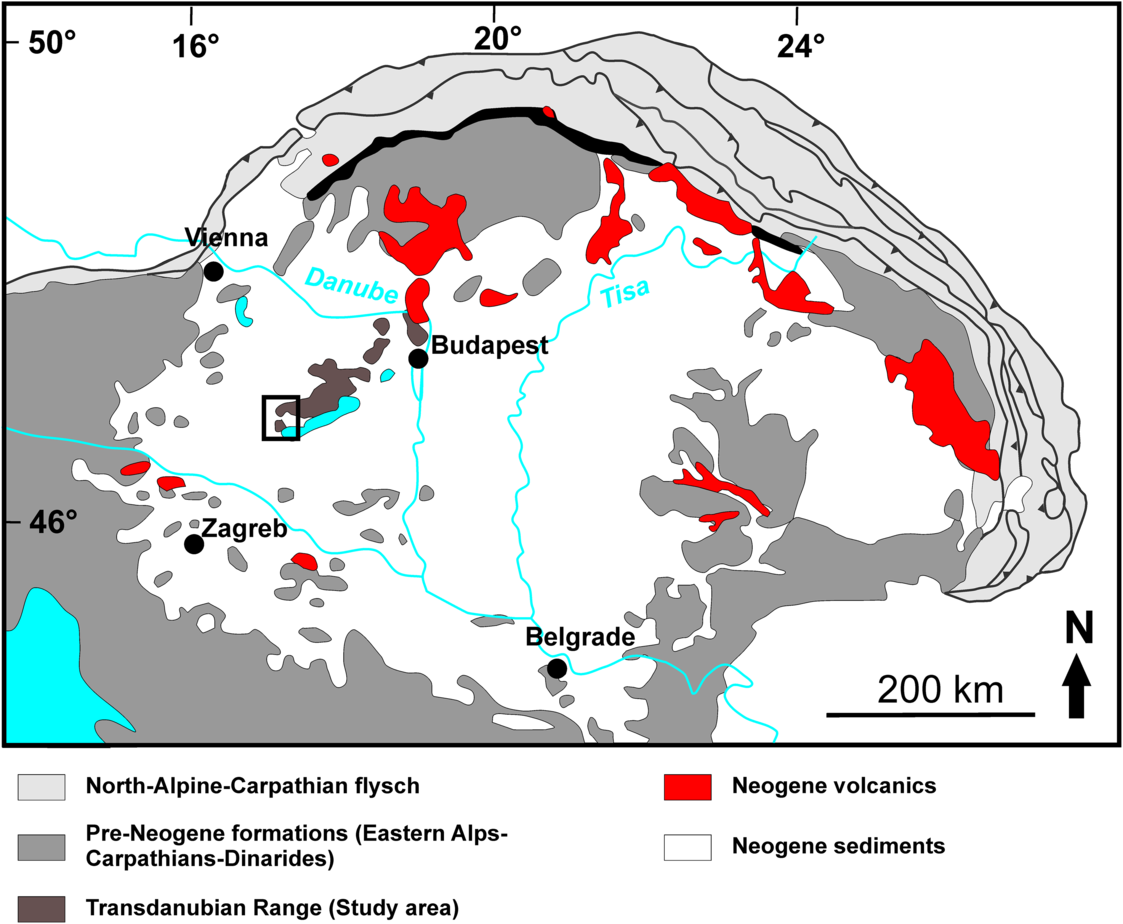
Fig. 1. The position of the study area within the Transdanubian Range in the western part of the Pannonian Basin System. Modified after Molnár et al. (Reference Molnár, Lukács, Dunkl, Schmitt, Kiss, Seghedi, Szepesi and Harangi2019). The black bracket indicates the study area.
The studied Cserszegtomaj Kaolin Formation (Bohn, Reference Bohn1979; Budai et al. Reference Budai, Császár, Csillag, Dudko, Koloszár, Majoros and Budai1999) is a yellow, grey terrestrial pelite filling some of the sinkholes in the KH and SBM (Fig. 2). The earliest reports considered this up to 100 m thick formation as a heteropic facies of the Eocene bauxites (Szentes, Reference Szentes1957; Csillag, Reference Csillag1959; Bárdossy, Reference Bárdossy1961), but later studies suggested a Miocene age based on a reworked Middle Eocene to Late Oligocene nannoplankton assemblage (Bohn, Reference Bohn1979).

Fig. 2. Simplified geological map of the study area and the locations of the Uzst-2 and Kht-4 boreholes in the Southern Bakony Mountains and Keszthely Hills (map base after Gyalog, Reference Gyalog2005).
This study provides an up-to-date description of the composition, provenance and age of the Cserszegtomaj Kaolin Formation using X-ray diffraction (XRD), heavy mineral analysis and zircon U–Pb geochronology. The results obtained from this highly altered terrestrial sediment trapped and preserved in sinkholes also proved to be useful in understanding the evolution of carbonate etchplain surfaces (cf. Thomas, Reference Thomas2016) in time and space characterized by intense erosion.
2. Geological setting
The TR is characterized by up to 3 km thick Triassic to Cretaceous marine sediments covering Variscan low-grade metamorphic rocks and Permian siliciclastics (Fig. 2; Haas, Reference Haas2013). The sedimentation of Mesozoic sequences has been controlled and interrupted by multiphase events of Cretaceous Eo-Alpine tectonics. The uplifted, subaerially exposed strata suffered erosion and karstification accompanied by the formation of Albian and Santonian bauxite deposits and their siliciclastic cover sequences (Mindszenty et al. Reference Mindszenty, Csoma, Török, Hips and Hertelendi2000). This terrestrial sedimentation was followed by marine sequences until an uplift event at the end of the Late Cretaceous, causing long-lasting denudation, karstification and etchplanation under tropical climatic conditions which lasted until the Middle Eocene. This subaerial exposure led finally to the formation of the Eocene bauxite (Csillag & Sebe, Reference Csillag, Sebe and Lóczy2015), which trapped airborne volcanic ash from the Periadriatic igneous activity (Dunkl, Reference Dunkl1992). Middle Eocene to Early Oligocene times were characterized by the development of the Slovenian–Hungarian Palaeogene Basin (Báldi, Reference Báldi1984; Fodor et al. Reference Fodor, Jelen, Márton, Skaberne, Čar and Vrabec1998). Between the Oligocene and Middle Miocene, the TR became detached from the Alpine realm by strike-slip faults and reached its present position (Balázs et al. Reference Balázs, Matenco, Magyar, Horváth and Cloetingh2016 and references therein). The large-scale horizontal movement and rotation triggered inversion of the Palaeogene basin, and generated erosion but also an accumulation of up to 800 m thick Oligocene to Lower Miocene, Alpine-derived molasse-type siliciclastic sediments that covered much of the Bakony Mountains (Korpás, Reference Korpás1981; Benedek et al. Reference Benedek, Nagy, Dunkl, Szabó and Józsa2001). By the end of this deposition event, the Upper Triassic carbonates became generally exposed in the TR. Most of the younger Mesozoic and early Palaeogene strata have been removed in the KH, while in the SBM their remnants are preserved until present times (Bohn, Reference Bohn1979; Budai et al. Reference Budai, Császár, Csillag, Dudko, Koloszár, Majoros and Budai1999). Karstification caused etchplanation on the Late Triassic carbonate plateaus while along major faults deep sinkholes developed and filled up with kaolin deposits with reworked Middle Eocene to Late Oligocene nannoplankton implying their Miocene age (Bohn, Reference Bohn1979). These sinkholes, despite being partly eroded, can reach ~50 m depth in the KH area and ~100 m in the SBM (Bohn, Reference Bohn1979).
Between 21 and 10 Ma, a massive ash-veneer produced by the Carpathian–Pannonian volcanism spread over the Eastern Alps, the northwestern Dinarides and the northeastern Southern Alps (e.g. Lukács et al. Reference Lukács, Harangi, Bachmann, Guillong, Danišík, Buret, von Quadt, Dunkl, Fodor, Sliwinski, Soós and Szepesi2015, Reference Lukács, Harangi, Guillong, Bachmann, Fodor, Buret, Dunkl, Sliwinski, von Quadt, Peytcheva and Zimmerer2018; Rocholl et al. Reference Rocholl, Schaltegger, Gilg, Wijbrans and Böhme2018). Thus, the TR became episodically covered with volcanic ash (e.g. Püspöki et al. Reference Püspöki, Kozák, Kovács-Pálffy, Földvári, McIntosh and Vincze2005). From Langhian to Serravallian times (16.5–11.5 Ma), the TR was dissected and the newly formed molasse-type basins became filled up with limestone in the KH area. In the SBM area, the basin filled sequentially with siliciclastic conglomerate, sandstone and limestone (Budai et al. Reference Budai, Császár, Csillag, Dudko, Koloszár, Majoros and Budai1999). Some of the eroded Cretaceous and Eocene bauxite deposits were resedimented as red clays (Tóth & Varga, Reference Tóth and Varga2014; Kelemen et al. Reference Kelemen, Dunkl, Csillag, Mindszenty, von Eynatten and Józsa2017). There is an ongoing debate as to whether the Middle Miocene Climatic Optimum (17–15 Ma; Zachos et al. Reference Zachos, Pagani, Sloan, Thomas and Billups2001) could have caused authigenic kaolinite formation in the red clays and in the kaolin deposits or if their kaolinite content was inherited from Cretaceous–Palaeogene red clays and bauxites (Schwarz, Reference Schwarz1997; Kelemen et al. Reference Kelemen, Dunkl, Csillag, Mindszenty, von Eynatten and Józsa2017).
3. Materials and Methods
Kaolin samples of ~500 g initial mass were collected from the vertical boreholes of Uzst-2 (top: 18–20 m; middle: 58–60 m; base: 106–107 m) and Kht-4 (4.7 m; Fig. 2). XRD analyses were carried out at the Institute for Geological and Geochemical Research of the Hungarian Academy of Sciences and the Eötvös Loránd University, Budapest. Kht-4 is dominated by grey and ochre-coloured clay and the Uzst-2 core divides into an upper, red, silty, and a lower, grey, clayey, pyrite-bearing section (Fig. 3).
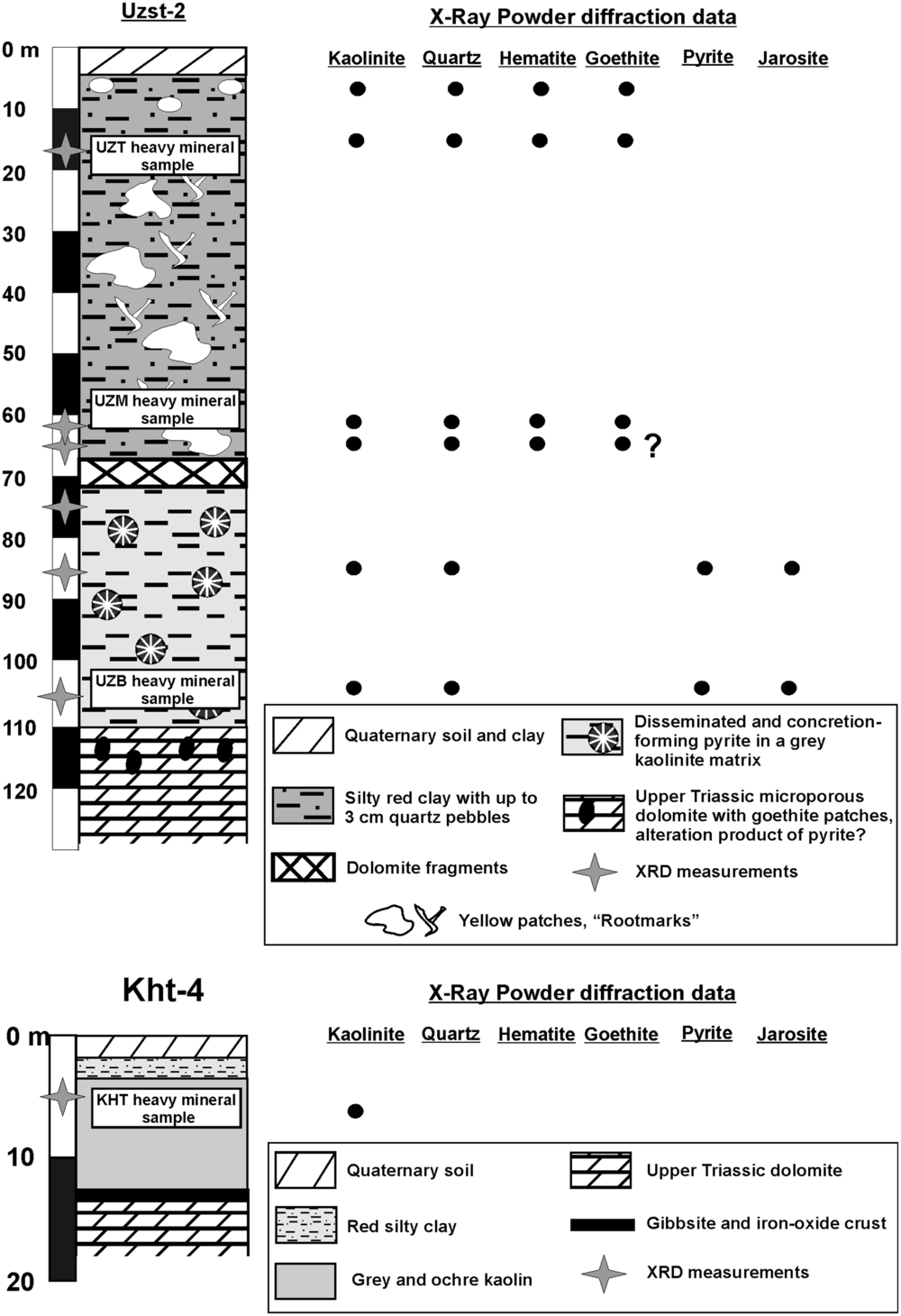
Fig. 3. Logs of Uzst-2 and Kht-4 boreholes combined with the results of qualitative X-ray powder diffraction phase analysis. Quartz and kaolinite are generally present at every level of Uzst-2. The iron-bearing phases show significant changes corresponding to the colour change from the red, goethite–hematite-dominated upper section to the grey, pyrite- and jarosite-bearing bottom section. The Kht-4 sample contains exclusively kaolinite. For detailed XRD spectra see Supplementary Figure S1 (available online at https://doi.org/10.1017/S0016756820000515).
For further analysis, samples were treated with warm-water (~50 °C) ultrasonic agitation and decantation to remove the loose clay matrix. The residual sand fraction yielded 0.002 wt % for the KH sample, ~0.1 wt % for the top/middle and 0.02 wt % for the base of the Uzst-2 core. Approximately 1 mm sized, well-rounded quartz grains are present in all Uzst-2 samples. The 63–125 μm sieve fractions were used for heavy mineral analysis and zircon U–Pb geochronology. Due to the limited amount of available detrital grains, after Na–polytungstate heavy liquid separation, all transparent detrital grains were handpicked and embedded in 1 inch (2.54 cm), polished epoxy mounts. These were used later for both heavy mineral identification and U–Pb zircon dating with the laser ablation–inductively coupled plasma–mass spectrometer (LA-ICP-MS) method. Zircon–tourmaline–rutile (ZTR) index values were calculated according to Hubert (Reference Hubert1962). LA-ICP-MS measurements were carried out at the Göchron Laboratories, Geoscience Center, University of Göttingen. Details of this technique are available in Kelemen et al. (Reference Kelemen, Dunkl, Csillag, Mindszenty, von Eynatten and Józsa2017) and references therein.
4. Results
4.a. X-ray diffraction data
The evaluated XRD diagrams are presented in Supplementary Figure S1 (available online at https://doi.org/10.1017/S0016756820000515). XRD analysis revealed only kaolinite in the Kht-4 sample, while in the Uzst-2 samples quartz and iron-bearing phases were identified along with kaolinite. In the upper, red part the dominant iron-bearing phases are goethite and hematite, while the lower, grey section contains mostly pyrite and some jarosite (Fig. 3).
4.b. Heavy mineral spectra
The ultra-stable minerals (zircon, tourmaline, rutile) dominate the heavy mineral fractions of all samples (Fig. 4). The Kht-4 sample has a ZTR index of ~96 %. Zircon is a major phase (~75 %), while rutile and tourmaline are about ~15 and ~5 %, respectively, and some other phases such as staurolite, amphibole, pyroxene and monazite were detected in trace amounts (<2 %). In borehole Uzst-2, all samples are dominated by a balanced zircon–rutile–tourmaline assemblage (ZTR values ~92–95 %), but minor amounts of kyanite, staurolite, amphibole and pyroxene are also present (<5 %) in the Uzst samples (Fig. 4). The ratio of euhedral zircon crystals to the total number of zircons is between 17 % and 42 %. Detailed heavy mineral data are presented in Supplementary Table S2 (available online at https://doi.org/10.1017/S0016756820000515).

Fig. 4. Heavy mineral composition of the Kht-4 and Uzst-2 samples. n = number of identified transparent/translucent heavy mineral grains. For details see Supplementary Table S2 (available online at https://doi.org/10.1017/S0016756820000515).
4.c. Zircon U–Pb ages
The U–Pb raw data are listed in Supplementary Table S3 (available online at https://doi.org/10.1017/S0016756820000515). In the Kht-4 sample, the majority of the ages are Miocene (89 %), ranging in a tight cluster between 18 and 14 Ma (Fig. 5). Some Permo-Triassic ages are also present (~6 %), along with a very few scattered Proterozoic to Eocene ages. The Uzst-2 samples show a more complex age spectrum. The Miocene ages (10–52 %) are between 20 and 16 Ma, significantly older than that of the Kht-4 sample (18–14 Ma; Fig. 5b). Palaeogene ages range between 44.1 and 29.3 Ma and form a significant group in two of the samples (top: 8 %, middle: 12 %), while all Uzst-2 samples show various ages from Proterozoic to Cretaceous (Fig. 5).
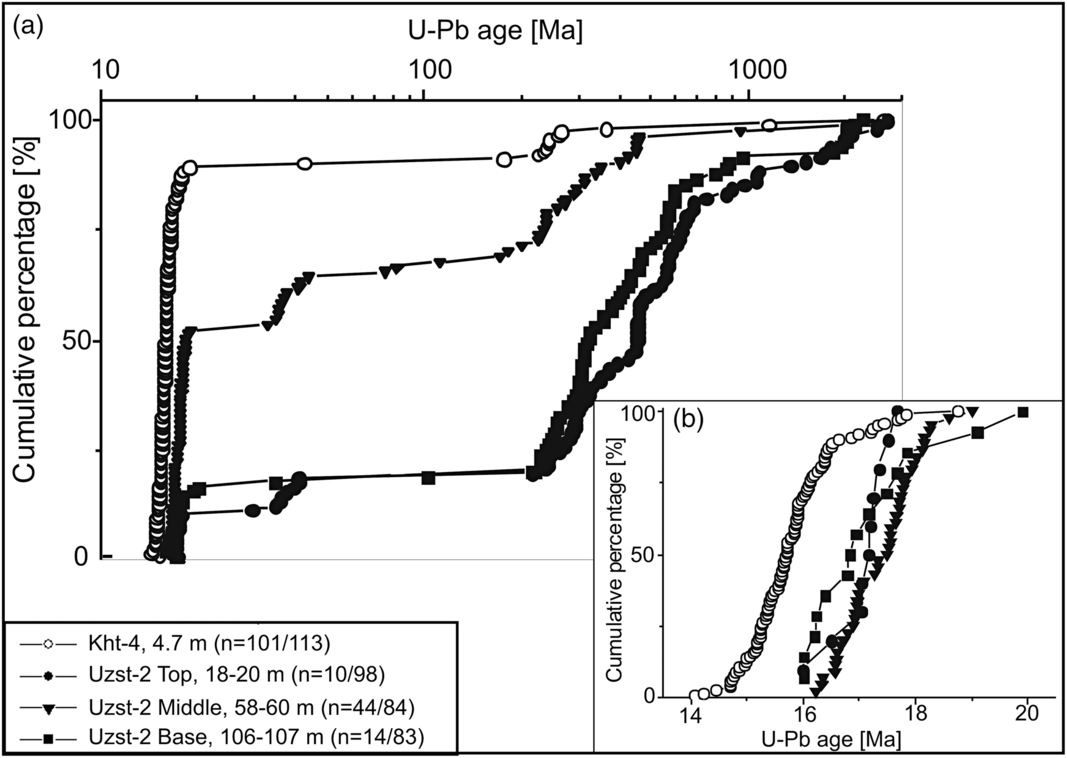
Fig. 5. (a) Cumulative plot of 90–110 % concordant detrital zircon U–Pb ages of the samples from the Kht-4 and Uzst-2 boreholes (Keszthely Hill and Southern Bakony Mountains). The Kht-4 sample has a high proportion of Miocene ages (89 %; 101 out of 113 respectively), while the Uzst-2 samples show much fewer Miocene ages (10–52 %) and contain significant amounts of Precambrian to Eocene ages. (b) The ages younger than 20 Ma are normalized to 100 % and plotted on a cumulative diagram insert. The individual zircon ages of all three Uzst-2 samples are mostly scattered within the 19–16 Ma time intervals, while the Kht-4 sample shows significantly younger ages and the data ranges of the two sampling locations have minor overlap. For more details see Supplementary Table S3 (available online at https://doi.org/10.1017/S0016756820000515).
5. Discussion
5.a. Provenance of the kaolin deposit in the Southern Bakony Mountains (Uzst-2 borehole)
The well-constrained age components (Supplementary Table S4 available online at https://doi.org/10.1017/S0016756820000515) identified by the procedures of Dunkl & Székely (Reference Dunkl and Székely2003) and Vermeesch (Reference Vermeesch2012) conform well to some of the major eruption phases of the Carpathian–Pannonian Neogene volcanism (Lukács et al. Reference Lukács, Harangi, Bachmann, Guillong, Danišík, Buret, von Quadt, Dunkl, Fodor, Sliwinski, Soós and Szepesi2015; Rocholl et al. Reference Rocholl, Schaltegger, Gilg, Wijbrans and Böhme2018). Thus, we propose that the eruptions at c. 17.8 ± 0.1 to 16.7 ± 0.2 Ma delivered the majority of Miocene zircons to the Uzst-2 samples (Fig. 6). The similarity in the Th/U ratio of the zircon crystals in the kaolin and in the ashes provides further evidence for the volcanogenic provenance of the youngest zircons (Fig. 7). The trace amounts of amphibole and pyroxene grains most likely had the same source. Two samples contain a significant amount of Eocene zircon crystals (means of the age components: 37.4 ± 0.4 to 36.7 ± 3.3 Ma). The pre-Cenozoic U–Pb age components (Fig. 6; Supplementary Table S4 available online at https://doi.org/10.1017/S0016756820000515) most likely derived from Proterozoic to Cadomian-, Ordovician- and Variscan igneous formations, as well as Permian and Middle Triassic volcanics of the TR (Fig. 6). These principal age components and the ultra-stable heavy mineral compositions are similar to those recorded in the Miocene red clays of the Bakony Mountains and Balaton Highlands, which are considered to reflect recycled Eocene and Cretaceous bauxite deposits (Kelemen et al. Reference Kelemen, Dunkl, Csillag, Mindszenty, von Eynatten and Józsa2017). This fact indicates that the Uzst-2 sinkhole most likely received recycled sediments from locally exposed Palaeogene sequences most probably by fluvial transportation (Fig. 8).

Fig. 6. Kernel density estimation (KDE) of zircon U–Pb age component analysis of the Uzst-2 and Kht-4 samples in the 1000–0 Ma time interval. See the mean values of the individual age components of the samples in Supplementary Table S4 (available online at https://doi.org/10.1017/S0016756820000515). Details of the KDE method are explained in Vermeesch (Reference Vermeesch2012). The most significant age components in all samples are related to the Carpathian–Pannonian Neogene volcanism. Easily detectable amounts of Palaeogene zircons are present in the top and middle samples of the Uzst-2 core. The Uzst-2 samples contain a significant amount of most likely inherited pre-Cenozoic zircons generated by the Late Proterozoic to Cadomian-, Ordovician- and Variscan igneous events, as well as Permian and ‘Pietra Verde’ volcanism.
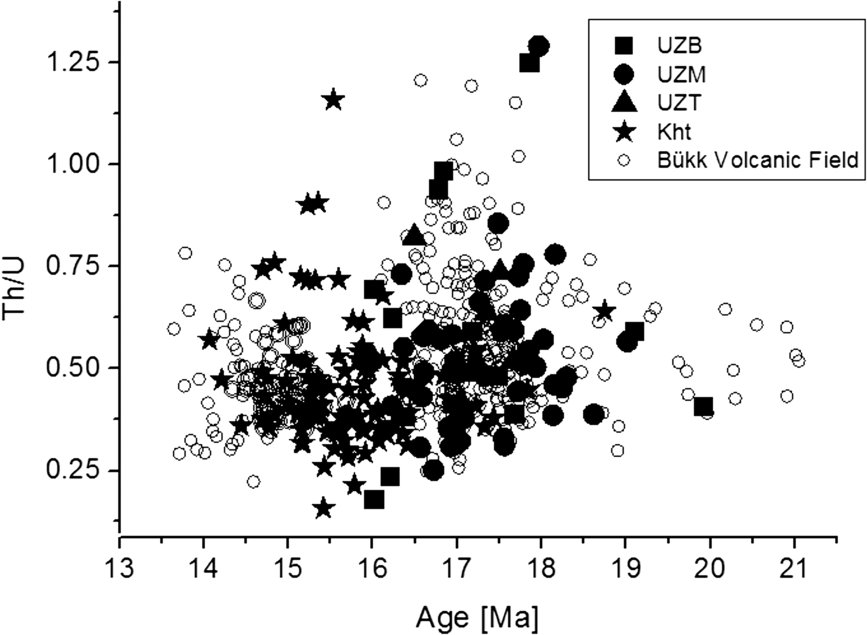
Fig. 7. Zircon U–Pb ages vs Th/U ratios from Kht-4 and Uzst-2 samples compared to Bükk Volcanic Field data (Lukács et al. Reference Lukács, Harangi, Guillong, Bachmann, Fodor, Buret, Dunkl, Sliwinski, von Quadt, Peytcheva and Zimmerer2018).
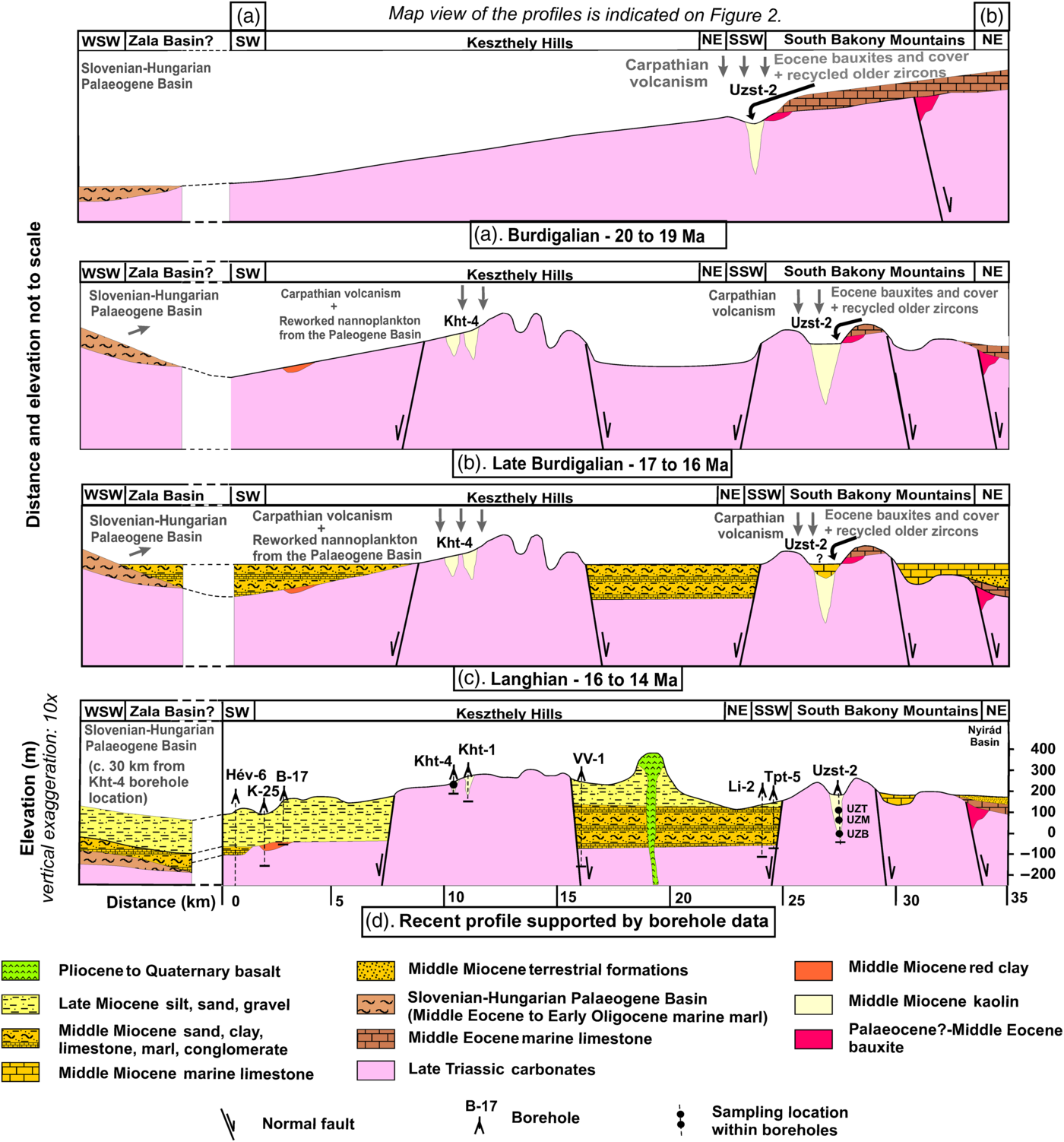
Fig. 8. Formation model of the kaolin deposits in four steps. (a) During Burdigalian times the Keszthely Hills and Southern Bakony Mountains formed a continuous, eroding etchplain. The Uzst-2 sinkhole accumulated sediments from the Eocene bauxites and their cover sequences as local sources, and from the Carpathian Neogene volcanism as a distant source. (b) During Late Burdigalian times the area is dissected by normal faults. The KH sinkholes started to develop and accumulate materials from the airborne volcanic ash of the Carpathian–Pannonian Neogene volcanism and windblown dust derived from the exposed Slovenian–Hungarian Palaeogene Basin. (c) During Langhian times, the Uzst-2 sinkhole was filled up and/or partly covered. (d) Idealized cross-section of the present geological situation of the study area, which served as a starting point for the reconstruction of the Burdigalian and Langhian models. Base maps: Budai et al. (Reference Budai, Császár, Csillag, Dudko, Koloszár, Majoros and Budai1999) and Gyalog (Reference Gyalog2005). Borehole data are acquired from the Hungarian Geological Reference Library. Sampling points are indicated by black dots. Boreholes, used to construct the profile, are located within a 4 km distance. Map view of the profiles is indicated in Figure 2.
5.b. Provenance of the kaolin deposit in the Keszthely Hills (Kht-4 borehole)
The extremely low sand content, zircon-dominated heavy mineral spectrum and predominant Miocene U–Pb ages suggest that the sediment in the Kht-4 sinkhole is mostly airborne in origin and altered in situ. This implies that the filling is mainly of tephra originating from the Carpathian–Pannonian Neogene volcanics, which provided the majority of zircons and some amphibole and pyroxene crystals. An additional admixture must have been windblown dust derived from the exposed Slovenian–Hungarian Palaeogene Basin which supplied nannoplankton, rutile, tourmaline, staurolite and monazite. The single Palaeogene zircon crystal has only limited significance as a provenance indicator. The Triassic zircons most likely derived from the “pietra verde” tuff intercalations of the Triassic sequence of the TR (Dunkl et al. Reference Dunkl, Farics, Józsa, Lukács, Haas and Budai2019).
5.c. Development and timing of the sinkholes
The development of the c. 50 and 100 m deep kaolin-bearing sinkholes in KH and SBM along with appropriate, tectonically controlled fracturing requires warm-humid climate conditions and an elevated topographic position above the karst water table (e.g. D’Argenio & Mindszenty, Reference D’Argenio and Mindszenty1995). The continuous age distribution between 20 and 16 Ma in the Uzst-2 samples (Fig. 5b) implies that the source materials of the red clay and kaolin assemblages in this area were deposited during and prior to the Miocene Climatic Optimum (17–15 Ma; Zachos et al. Reference Zachos, Pagani, Sloan, Thomas and Billups2001). The dominance of Miocene ages and the almost total absence of Palaeogene ages in the Kht-4 sinkhole suggest that kaolin deposits in the KH area accumulated soon after the formation of their hosting sinkholes, or both the sinkhole formation and filling events occurred contemporaneously, in agreement with Csillag & Sebe (Reference Csillag, Sebe and Lóczy2015). The shift between the Miocene ages of the SBM and KH samples suggests that by c. 16 Ma the Uzst-2 sinkhole had already been filled, thus could not accommodate more airborne material, and became covered by Middle Miocene sediments deposited after a horst- and graben-forming tectonic event (Csillag & Nádor, Reference Csillag and Nádor1997; Fig. 8b, c). Meanwhile the Kht-4 sinkhole was still exposed and accumulated material until 15–14 Ma. The in situ transformation into kaolin likely occurred during the Middle Miocene Climatic Optimum (Zachos et al. Reference Zachos, Pagani, Sloan, Thomas and Billups2001) under at least subtropical warm and humid climatic conditions dominating the Pannonian Basin during this time interval (Jiménez-Moreno, Reference Jiménez-Moreno2007).
Bauxites and bauxitic to kaolinitic red clays were reported from the Early to the Middle Miocene, from several localities in the wider surroundings of the Pannonian Basin and across Europe. Wagreich et al. (Reference Wagreich, Zetter, Bryda and Peresson1997) mentioned gibbsitic karst bauxites from the Hieflau area in the Northern Calcareous Alps. Further north, a 50 m thick bauxitic laterite profile was described on the Miocene Vogelsberg continental basalt lavas palaeolattitude 45 by Schwarz (Reference Schwarz1997). Subaerial exposure in the Apennines and Dinarides also resulted in bauxitic (ferrallitic) weathering products probably related to the Middle Miocene Climatic Optimum (Kici et al., Reference Kici, Peza, Skhupi and Xhomo1991; Simone et al., Reference Simone, Carannante, D’Argenio, Ruberti and Mindszenty1991; Šinkoveć & Šakać, Reference Šinkoveć and Šakać1991).
6. Conclusions
The XRD, heavy mineral and U–Pb age data reveal major differences of the Keszthely Hills and Southern Bakony Mountains regarding the source of their kaolin deposits, mainly because they developed during different time intervals (Fig. 8a–d).
The major sources of the Uzst-2 sinkhole (Southern Bakony Mountains) are fluvially resedimented local Eocene bauxites with their Palaeogene cover sequences and airborne ash from the distal Carpathian–Pannonian Neogene volcanism. The strongly altered parent materials were transported into a pre-existing, deep sinkhole between 20 and 16 Ma.
The Kht-4 sinkhole (Keszthely Hills) hosts a different assemblage of airborne origin. The most important source is from the Carpathian–Pannonian Neogene volcanism. The airborne volcanic ash trapped in newly formed sinkholes and underwent in situ weathering during the Middle Miocene Climatic Optimum. The presence of Palaeogene nannoplankton assemblages indicates that this site also received redeposited material from the inverted Slovenian–Hungarian Palaeogene Basin.
Formation processes were controlled by the tectonic evolution of the surrounding landscape. Uplift between 18 and 14 Ma triggered karstification and formation of kaolin deposit in the Kht-4 sinkhole under the warm and humid conditions of the Miocene Climatic Optimum. This study confirms that heavy mineral studies combined with single-crystal dating of detrital grains may be particularly useful to reconstruct stages of landform evolution and denudational history of tectonically affected planation surfaces.
Acknowledgements
This study was funded by the Hungarian Scientific Research Fund (ID: K106197), the ERASMUS+ Internship program (contract number: 18/1/KA103/047073/SMP) and Papp Simon Foundations. The authors are grateful to Andreas Kronz, Judit Nagy, Irina Ottenbacher, Cornelia Friedrich, László Szikszay and Zoltán Lantos for help with analytical and documentation procedures. Special thanks to László Fodor, Réka Lukács and Szabolcs Harangi for helpful discussions.
Supplementary material
To view supplementary material for this article, please visit https://doi.org/10.1017/S0016756820000515