1. Introduction
The term ‘fault’ is derived from French faute (opening, gap, failure or flaw) and was first systematically treated as such by Margerie and Heim (Reference Margerie and Heim1888), later becoming one of the most important elements in geological structural analysis. What makes faults particularly important is that, once formed, they tend to become the loci of permanent weakness in the crust and, whenever favourably oriented with regard to the stress field, may localize significant strain increments (e.g. Holdsworth et al. Reference Holdsworth, Butler and Roberts1997; Naliboff et al. Reference Naliboff, Buiter, Péron-Pinvidic, Osmundsen and Tetreault2017). This observation is particularly evident in geologically old terranes where faults may have been reactivated multiple times (e.g. Braathen et al. Reference Braathen, Osmundsen and Gabrielsen2004; Redfield & Osmundsen, Reference Redfield and Osmundsen2009; Viola et al. Reference Viola, Scheiber, Fredin, Zwingmann, Margreth and Knies2016; Aldega et al. Reference Aldega, Viola, Casas-Sainz, Marcén, Román-Berdiel and van der Lelij2019; Kemp et al. Reference Kemp, Gillespie, Leslie, Zwingmann and Campbell2019). Some faults record activity that is separated by hundreds of millions of years (e.g. Torgersen et al. Reference Torgersen, Viola, Zwingmann and Harris2015 a; Nordbäck et al. Reference Nordbäck, Mattila, Zwingmann and Viola2022), which demonstrates that faults can control crustal strengths over very long timescales even though they are inactive at subcritical stress conditions for most of the time. This long-lived strength reduction is partly a consequence of the loss of cohesion along faults (Sibson, Reference Sibson1985), but more significantly due to the anomalously low friction coefficients of clay minerals and phyllosilicates that commonly make up the fault cores (e.g. Haines et al. Reference Haines, Marone and Saffer2014; Collettini et al. Reference Collettini, Tesei, Scuderi, Carpenter and Viti2019). Combined mechanical comminution and fluid–rock interactions cause the formation of interconnected clay/phyllosilicate-decorated slip surfaces or foliation planes, which appears to be the primary process causing progressive strain weakening in large fault zones (Collettini et al. Reference Collettini, Niemeijer, Viti and Marone2009; Haines et al. Reference Haines, Kaproth, Marone, Saffer and van der Pluijm2013; Torgersen & Viola, Reference Torgersen and Viola2014). Whereas this process may readily explain why pre-existing clay/phyllosilicate-rich faults are primed for repeated brittle reactivations, it does not fully account for how brittle faults can localize into and exploit pre-existing plastic shear zones. This type of structural superposition is observed along many major deformation zones (e.g. Braathen et al. Reference Braathen, Osmundsen and Gabrielsen2004; Ferreira et al. Reference Ferreira, Bezerra, Sousa, do Nascimento, Sá and França2008; Salomon et al. Reference Salomon, Koehn and Passchier2015; Scheiber et al. Reference Scheiber, Viola, Bingen, Peters and Solli2015; Gabrielsen et al. Reference Gabrielsen, Olesen, Braathen, Faleide, Baranwal and Lindholm2019), and offshore seismic studies show that basement-hosted plastic shear zones control fault localization and magnitude in the unconformably overlaying sedimentary rocks (e.g. Phillips et al. Reference Phillips, Jackson, Bell and Duffy2018; Osagiede et al. Reference Osagiede, Rotevatn, Gawthorpe, Kristensen, Jackson and Marsh2020). Pre-existing plastic shear zones may even impact on the entire architecture and geometry of rifted margins (e.g. Morley et al. Reference Morley, Haranya, Phoosongsee, Pongwapee, Kornsawan and Wonganan2004; Fossen et al. Reference Fossen, Khani, Faleide, Ksienzyk and Dunlap2017; Osmundsen & Péron-Pinvidic, Reference Osmundsen and Péron-Pinvidic2018; Kalani et al. Reference Kalani, Faleide and Gabrielsen2020; Osmundsen et al. Reference Osmundsen, Péron-Pinvidic and Bunkholt2021).
The superposition of brittle deformation on plastic shear zones is frequently observed along major low-angle detachment zones (e.g. Norton, Reference Norton1986; Andersen & Jamtveit, Reference Andersen and Jamtveit1990; Fossen, Reference Fossen1992; Osmundsen et al. Reference Osmundsen, Eide, Haabesland, Roberts, Andersen, Kendrick, Bingen, Braathen and Redfield2006). These often demonstrate a continuum of deformation rocks, reflecting the progressive development during semi-continuous exhumation through the brittle–plastic transition (Davis et al. Reference Davis, Darby, Yadong and Spell2002; Braathen et al. Reference Braathen, Osmundsen and Gabrielsen2004). In such a situation, brittle deformation is closely spaced in time with visco-plastic deformation and it is suggested that the transition between the two is controlled by ingress of fluids and reaction-driven mineralogical (and rheological) changes (Selverstone et al. Reference Selverstone, Axen and Luther2012). As such, one may question whether a progressive exhumation is always required for brittle deformation to localize along plastic shear zones, or if other mechanisms may lead to brittle reactivation that is kinematically and temporally distant.
If one only considers the frictional properties of plastic shear zones, it appears counter-intuitive that brittle faults should localize along such structures because the fine-grained, dynamically recrystallized mylonites that commonly make up the shear zones should have at least as high a frictional coefficient as the surrounding wall rocks. Mechanisms such as post-tectonic alteration-weakening (Backeberg et al. Reference Backeberg, Rowe and Barshi2016), strength anisotropy associated with the mylonitic/phyllonitic fabric (e.g. Crider, Reference Crider2015; Bolognesi & Bistacchi, Reference Bolognesi and Bistacchi2016; Samsu et al. Reference Samsu, Cruden, Molnar and Weinberg2021) and stiffness contrasts combined with grain-size reduction and mineral alteration (e.g. Jefferies et al. Reference Jefferies, Holdsworth, Wibberley, Shimamoto, Spiers, Niemeijer and Lloyd2006) have been proposed to explain this behaviour.
To improve the understanding of how brittle deformation may repeatedly localize along existing plastic shear zones, we have studied the Himdalen Fault in southeast Norway (Fig. 1), which is formally defined and described for the first time in this paper. The fault is associated with a strong topographic lineament that parallels the surrounding mylonitic fabric of the Ørje Shear Zone, a Meso- to Neoproterozoic orogen-scale high-strain zone associated with the development of the Sveconorwegian orogeny in southern Norway and Sweden (Fig. 1; Skjernaa, Reference Skjernaa1972; Viola et al. Reference Viola, Henderson, Bingen and Hendriks2011; Bingen et al. Reference Bingen, Viola, Möller, Vander Auwera, Laurent and Yi2021). Collectively, the herein termed Himdalen–Ørje Deformation Zone (HØDZ) is defined by a series of deformational products ranging from mylonite and cataclasite to pseudotachylite, breccia and gouge following the combined extent of the Himdalen Fault and the Ørje Shear Zone. The mylonitic component has been attributed to Sveconorwegian top-to-the-SE contractional deformation and subsequent post-orogenic top-to-the-W extensional reactivation (Viola et al. Reference Viola, Henderson, Bingen and Hendriks2011). Apart from these structural inferences, no radiometric ages are yet available for any of these deformational products, which prevents us from organizing the deformation zone into a correct time frame and understanding the superposition of brittle on visco-plastic deformation products.

Fig. 1. (a) Geological framework of southwestern Scandinavia. Large structures (in italics): MZ: Mylonite Zone; DT: Dalsland Boundary Thrust / Göta Älv Shear Zone / Lerdal Shear Zone; KP: Kristiansand–Porsgrunn Shear Zone; SS: Saggrenda–Sokna Shear Zone; LD: Listafjorden–Drangedal Fault Complex; NS: Nesodden Fault; HØDZ. Himdalen–Ørje Deformation Zone. (b) Bedrock map (Norges Geologiske Undersøkelse, 2021) of the wider Himdalen region showing the position of the Ørje Shear Zone and the Himdalen Fault. Stereonet plot shows foliation (as poles and contours), fold axis and mineral lineations within the Ørje Shear Zone as presented on published geological maps. (c) Hillshade (light source from NW) showing the Himdalen topolineament and the location of the two structural profiles in Figure 2.
In this paper we aim to constrain the structural history of the HØDZ by combining mapping of its structural architecture and deformation products with K–Ar fault gouge dating and 40Ar–39Ar geochronology of pseudotachylite veins, and of K-feldspar and white mica in the mylonites. We focus on constraining the timing of brittle reactivation along the Himdalen Fault, which may potentially be hundreds of millions of years younger than the assumed Meso- to Neoproterozoic mylonitic Ørje Shear Zone. The results provide new insights into the structural evolution of a long-lived plastic-to-brittle deformation zone and the mechanisms that control repeated structural reactivations. The study also demonstrates the use and challenges of illite crystallinity in interpreting multi-reactivated fault gouges. The new ages provide important novel constraints on the timing of the tectonic development of southern Norway and Sweden, as well as the offshore Skagerrak graben and potentially the Sorgenfrei–Tornquist zone.
2. Geological setting
The HØDZ is situated in SE Norway within the Sveconorwegian domain of the Precambrian basement in southern Norway and Sweden (Gaál & Gorbatschev, Reference Gaál and Gorbatschev1987; Andersen, Reference Andersen2005; Bingen et al. Reference Bingen, Nordgulen and Viola2008 b, Reference Bingen, Viola, Möller, Vander Auwera, Laurent and Yi2021; Slagstad et al. Reference Slagstad, Roberts, Marker, Røhr and Schiellerup2013; Fig. 1). The Sveconorwegian orogeny took place in the late Mesoproterozoic to early Neoproterozoic during a hot, long-duration continental collision between proto-Baltica and another plate, possibly Amazonia (Bingen et al. Reference Bingen, Viola, Möller, Vander Auwera, Laurent and Yi2021). The rocks within the Sveconorwegian domain are affected by numerous generally NNW–SSE-striking crustal-scale shear zones separating different lithotectonic units, previously termed sectors, segments (Berthelsen, Reference Berthelsen, Cogne and Slansky1980; Starmer, Reference Starmer1996), terranes (e.g. Bingen et al. Reference Bingen, Nordgulen and Viola2008 b; Viola et al. Reference Viola, Henderson, Bingen and Hendriks2011) and blocks (Andersen, Reference Andersen2005). The Scandinavian Deformation Front / Protogene Zone, the Mylonite Zone and the Dalsland Boundary Thrust / Göta Älv Shear Zone / Lerdal Shear Zone / Ørje Shear Zone (Berthelsen, Reference Berthelsen, Cogne and Slansky1980; Park et al. Reference Park, Åhäll and Bland1991; Stephens et al. Reference Stephens, Wahlgren, Weijermars and Cruden1996) are the most conspicuous shear zones east of the Oslo Rift, whereas the Kristiansand–Porsgrunn and Saggrenda–Sokna Shear Zones separate the Bamble and Kongsberg Lithotectonic Units, respectively, from the Telemarkia Lithotectonic Unit to the west (Mulch et al. Reference Mulch, Cosca, Andresen and Fiebig2005; Scheiber et al. Reference Scheiber, Viola, Bingen, Peters and Solli2015; Fig. 1a). Each block is, however, further dissected by internal high-strain zones (mylonites sometimes overprinted by cataclasites) branching out from the main shear zones (e.g. Park et al. Reference Park, Åhäll and Bland1991; Andersen, Reference Andersen2005; Bingen & Viola, Reference Bingen and Viola2018). The peak metamorphism in the Idefjorden Lithotectonic Unit east of the Oslofjord is dated to 990 Ma (eclogite facies; Munz et al. Reference Munz, Wayne and Austrheim1994; Möller et al. Reference Möller, Andersson, Dyck and Lundin2015; Pinán-Llamas et al. Reference Pinán-Llamas, Andersson, Möller, Johansson and Hansen2015; Möller & Andersson, Reference Möller and Andersson2018) and between 1140 and 1075 Ma in the Kongsberg and Bamble Lithotectonic Units to the west (granulite facies; Andersen & Grorud, Reference Andersen and Grorud1998; Bingen et al. Reference Bingen, Birkeland, Nordgulen and Sigmond2001, Reference Bingen, Davis, Hamilton, Engvik, Stein, Skar and Nordgulen2008 a; Bingen & Viola, Reference Bingen and Viola2018).
Dedicated chronological data for the timing of shear zones in the Sveconorwegian domain are generally less common and precise. One sample taken close to the Göta Älv shear zone gave an age of 974 ± 22 for the outer rim of a zircon (Ahlin et al. Reference Ahlin, Hegardt and Cornell2006), and the maximum age of metamorphism (amphibolite facies) is in the range 910–990 Ma (Park et al. Reference Park, Åhäll and Bland1991; Stephens et al. Reference Stephens, Wahlgren, Weijermars and Cruden1996 and references therein). U–Pb-dating of titanite in the Mylonite Zone yielded a concordant age of c. 920 Ma, believed to represent the amphibolite metamorphism in the zone (Johansson & Johansson, Reference Johansson and Johansson1993), whereas Wahlgren & Kähr (Reference Wahlgren and Kähr1977) dated a post-tectonic lamprophyre dyke crossing the Mylonite Zone to 904 ± 13 Ma (whole-rock K–Ar), representing a minimum age for the visco-plastic deformation there. 40Ar–39Ar biotite and white mica ages from greenschist-facies, extension-related shear fabric of the Mylonite Zone range between 922 and 860 Ma (Viola et al. Reference Viola, Henderson, Bingen and Hendriks2011), which is a time interval associated with rapid cooling in the whole eastern part of the orogen (Ulmius et al. Reference Ulmius, Möller, Page, Johansson and Ganerød2018).
West of the Oslofjord, top-to-the-NW contractional displacement associated with the Kristiansand–Porsgrunn Shear Zone is constrained by the Morkheia monzonite suite and the Hovdefjell–Vegårdshei augen gneiss, giving a maximum age between 1132 ± 3 and 1140 ± 13 Ma (Heaman & Smalley, Reference Heaman and Smalley1994; Bingen & Viola, Reference Bingen and Viola2018). In one locality west of Lake Væteren in the Kongsberg Lithotectonic Unit, a post-mylonite lamprophyre dyke was dated to 1033 ± 12 Ma (zircon U–Pb), giving a minimum age for this deformation (Bingen & Viola, Reference Bingen and Viola2018). Late overprinting extensional fabrics of the Kristiansand–Porsgrunn Shear Zone are dated to 891 ± 3 Ma by 40Ar–39Ar geochronology of synkinematic muscovite (Mulch et al. Reference Mulch, Cosca, Andresen and Fiebig2005).
Some of the N–S- to NNE–SSW-striking master shear zones that constrain the Proterozoic Idefjorden Lithotectonic Unit in the east and the Kongsberg and Bamble Lithotectonic Units in the west merge with the master faults of the Permian Oslo Graben. These faults display complex structural relations and are characterized by shifting strain, P–T conditions and different types of fault rocks including protocataclasite, cataclasite, ultracataclasite and fluid breccia (e.g. Swensson, Reference Swensson1990; Olaussen et al. Reference Olaussen, Larsen and Steel1994; Larsen et al. Reference Larsen, Olaussen, Sundvoll and Heeremans2008 a, b; Torgersen et al. Reference Torgersen, Viola, Zwingmann and Henderson2015 b; Gabrielsen et al. Reference Gabrielsen, Nystuen and Olesen2018). Particularly in the northern extension of these faults (north of the northernmost segment of the Oslo Graben) mylonitic rocks are occasionally found in the core of these faults (Gabrielsen et al. Reference Gabrielsen, Nystuen and Olesen2018).
Several complex and reactivated fault systems in south-central and south Norway are characterized by both visco-plastic and brittle deformation rocks, some of which are composed of fault gouge reaching thicknesses up to tens of metres (e.g. the Sirdal and Listafjorden–Drangedal fault complexes; Gabrielsen et al. Reference Gabrielsen, Olesen, Braathen, Faleide, Baranwal and Lindholm2019). Anticipated or documented Permian (or younger) overprint occurs only in some of the shear zone segments. The youngest brittle reactivation in the ‘Great Friction Breccia’ associated with the Saggrenda–Sokna shear zone (Bugge, Reference Bugge1936; Starmer, Reference Starmer, Tobi and Touret1985; Scheiber et al. Reference Scheiber, Viola, Bingen, Peters and Solli2015; Bingen & Viola, Reference Bingen and Viola2018) has a probable Permian–Triassic age (Larsen et al. Reference Larsen, Olaussen, Sundvoll, Heeremans, Ramberg, Bryhni and Nøttvedt2008 b; Scheiber et al. Reference Scheiber, Viola, Bingen, Peters and Solli2015; Torgersen et al. Reference Torgersen, Viola, Zwingmann and Henderson2015 b). This is also the case for the N–S-striking Nesodden fault, which is characterized by multistage ductile deformation with post-Caledonian (Carboniferous–Permian?) brittle fault rocks (e.g. Swensson, Reference Swensson1990; Ganerød et al. Reference Ganerød, Braathen and Willemoes-Wissing2008). Also several other faults north of the Oslo area in the Oslo–Trondheim lineament zone (Gabrielsen & Ramberg, Reference Gabrielsen and Ramberg1979; Gabrielsen & Braathen, Reference Gabrielsen and Braathen2014), mainly comprising N–S-striking normal faults, display multiphase deformation with visco-plastic (Proterozoic?) and brittle (Carboniferous–post-Permian?) deformation rocks (see Gabrielsen et al. Reference Gabrielsen, Nystuen and Olesen2018 for summary). The presence of often thick accumulation of fault gouge in many of these faults has been used to infer Mesozoic or even Cenozoic reactivation histories (e.g. Gabrielsen et al. Reference Gabrielsen, Nystuen and Olesen2018). Indeed, Mesozoic to recent fault activity has been recorded in the major fault systems of coastal south Norway (Eide et al. Reference Eide, Torsvik and Andersen1997; Fossen et al. Reference Fossen, Mangerud, Hesthammer, Bugge and Gabrielsen1997; Ksienzyk et al. Reference Ksienzyk, Wemmer, Jacobs, Fossen, Schomberg, Süssenberger, Lünsdorf and Bastesen2016; Fossen et al. Reference Fossen, Khani, Faleide, Ksienzyk and Dunlap2017; Hestnes et al. Reference Hestnes, Gasser, Scheiber, Jacobs, van der Lelij, Schönenberger and Ksienzyk2022) and in the fault systems in the Kongsberg Lithotectonic Unit (Torgersen et al. Reference Torgersen, Viola, Zwingmann and Henderson2015 b).
In summary, age data from mylonitic shear zones in the Sveconorwegian domain display a Sveconorwegian age signature within the timespan c. 1050–1033 Ma (Bingen & Viola, Reference Bingen and Viola2018). A greenschist facies reactivation of at least some of the shear zones (e.g. the Dalsland Boundary Thrust and the Kristiansand–Porsgrunn Shear Zone) occurred during late stages of the Sveconorwegian orogeny at c. 920–890 Ma (Mulch et al. Reference Mulch, Cosca, Andresen and Fiebig2005). Many of these structures are overprinted by brittle deformation developed concurrently with the border faults of the Permian Oslo Rift or during the Mesozoic subsidence of the Oslo Graben (Torgersen et al. Reference Torgersen, Viola, Zwingmann and Henderson2015 b).
2.a. The Himdalen–Ørje Deformation Zone
The Himdalen–Ørje Deformation Zone (HØDZ) is a collective term for the 1–2 km wide NW–SE-trending deformation zone that comprises the Ørje Shear Zone and the Himdalen Fault east of the Oslo Rift (Fig. 1). The Ørje Shear Zone coincides with the eastern margin of the Kongeberg–Marstrand block of Andersen (Reference Andersen2005) and is positioned inside the Idefjorden Lithotectonic Unit (Skjernaa, Reference Skjernaa1972; Bingen et al. Reference Bingen, Birkeland, Nordgulen and Sigmond2001, Reference Bingen, Nordgulen and Viola2008 b). It is a high-strain arch with an average orientation of its mylonitic foliation of 240/45, stretching >100 km from Lillestrøm in the northwest to Aremark in the south (Fig. 1b). Fold axis and mineral lineation measurements within the Ørje Shear Zone, available in published bedrock maps, plunge overall NW and SE, with a spread towards E–W orientations (Fig. 1b). Viola et al. (Reference Viola, Henderson, Bingen and Hendriks2011) observed that an early high-grade fabric associated with top-to-the SE compression is partly reworked by later lower-grade (greenschist facies) top-to-the-W extensional shearing.
The Ørje Shear Zone has been traced southwards into the Grann zone (Hageskov, Reference Hageskov1980), which is a local equivalent to the Dalsland boundary thrust (Berthelsen, Reference Berthelsen, Cogne and Slansky1980; Gorbatschev, Reference Gorbatschev1988; Park et al. Reference Park, Åhäll and Bland1991) and is accordingly regarded as a part of the Dalsland boundary thrust and the Lerdal zone system (Berthelsen, Reference Berthelsen, Cogne and Slansky1980; Park et al. Reference Park, Åhäll and Bland1991). The latter is described as an imbricate stack of shear zones dipping 20–40° to the W and with a top-to-the-E displacement (Park et al. Reference Park, Åhäll and Bland1991). The eastern flank of the imbricate stack records extensional reactivation at lower-grade conditions and top-to-the-W sense of shear.
The Himdalen Fault is a previously unnamed section within the Ørje Shear Zone (‘Ørje crush zone’ of Skjernaa, Reference Skjernaa1972) defined by a clear brittle overprint and a distinct topographic expression (Fig. 1c). Skjernaa (Reference Skjernaa1972) states that ‘crush zone’ is an appropriate characterization ‘because real mylonites are uncommon’, although mylonite gneiss, blastomylonite, phyllonite and ‘hartschiefer’ (ultramylonite) have previously been described along the continuation of the zone (Quensel, Reference Quensel1916; Skjernaa, Reference Skjernaa1972). Our mapping indicates that the Himdalen section contains a plethora of mylonite gneisses overprinted by a sequence of brittle deformation products.
3. Architecture of the Himdalen–Ørje Deformation Zone
In the Himdalen study area, the HØDZ is characterized by a several tens of metres thick zone of deformation rocks including blastomylonite, mylonite, cataclasite and fault breccia and gouge. The zone varies in thickness, deformation style and intensity along its strike. The Himdalen Fault is traceable in the landscape as a NW–SE-striking topographic lineament (Fig. 1c) with a relief in the order of 100 m, that approximately follows the southwestern, hanging-wall margin of the Ørje Shear Zone (avg. mylonitic foliation dipdir/dip: 240/45). The Himdalen Fault dips moderately to the SW (225/45) and has sharp undulating contacts with the surrounding highly fractured to cataclastic granitic mylonites. It interferes with a NNE–SSW-striking pattern of topographic lineaments, some of which occur only on its NE margin, possibly representing fault splays (Fig. 1c). Normal, top-to-the-SW kinematic is inferred based on the presence of steep second-order fractures (230/70) interpreted as synthetic Riedel shears (Fig. 4f further below).
The architecture of the HØDZ is here presented in two structural profiles along the SW slope of the Himdalen lineament (Figs 1c, 2).

Fig. 2. Structural profiles across the Himdalen–Ørje Deformation Zone with location of geochronological samples. Profile 1 at UTM33N 6633204 293539, profile 2 at UTM33N 6632564 293914.
Profile 1 displays a more than 20 m thick zone dominated by rock lenses of granitic gneiss of moderate to intense deformation (Fig. 3a). The lowest part of the exposure comprises a protomylonitic granitic gneiss that is intensely overprinted by brittle deformation, and structurally overlain and cut by fault gouge and unconsolidated breccia. The gouge and breccia are partly covered by and intermingled with coarse to fine erosional deposits; however, ‘windows’ in the unconsolidated deposits display sheared and intensely fractured granitic gneiss verging into open (unconsolidated) breccia and fault gouge. These windows are interpreted as parts of the fault footwall and likely represent large fragments or lenses embedded in the fault gouge/unconsolidated breccia.

Fig. 3. Field, hand-specimen and thin-section photos of typical deformation rocks in the HØDZ at profile 1, UTM33N 6633204 293539. (a) Overview photograph of the lower portion of profile 1. (b, c) Ultramylonitic band in granitic mylonite with thin cross-cutting pseudotachylite veins (sample RG16-119A2). (c) Photomicrograph (plane polarized) of one of the thin veins in (b), possibly representing a recrystallized pseudotachylite.
A sharp border separates the fault gouge / unconsolidated breccia from a 1.5 m thick moderately to strongly sheared gneiss sequence above it (Fig. 3a–c). The lowermost 80 cm of the gneiss sequence, a protomylonitic gneiss with lens-shaped protoclasts, is separated by mm-thick sub-horizontal dark shear lozenge-shaped rock fragments. The rock is cross-cut by dark planar to irregular veinlets which are interpreted as pseudotachylite and/or ultracataclasite veins (Fig. 3c). This rock grades into a banded granitic gneiss overprinted by arrays of a 0.1–1 cm thick grey chequerboard pattern of quartz veins, defined by high-angle veins and sills.
A c. 30 cm thick band of biotite-rich dark gneiss and augen gneiss separates the granitic protomylonite sequence from another less intensely deformed granitic unit above it. The biotite-gneiss/augen-gneiss zone is interpreted as a recrystallized high-strain zone that possibly consisted of a phyllonite–blastomylonite sequence. It is structurally overlain by a more than 3 m thick unit of medium- to coarse-grained granitic blastomylonite that encompasses an aplitic, massive unit, which may represent a sheared (?syntectonic) granitic sill. The granitic blastomylonite also contains a 30 cm thick sequence of medium-grained biotite gneiss, recrystallized blastomylonite and a strongly sheared basic rock. The latter presumably is a sheared basic sill.
Profile 2 is situated c. 500 m NW of profile 1 (Figs. 2, 4). The profile has much in common with profile 1, albeit with differences in thickness of the units, probably due to the fault architecture of the master fault, which is characterized by lozenge-shaped rock bodies that have restricted along-strike continuity.
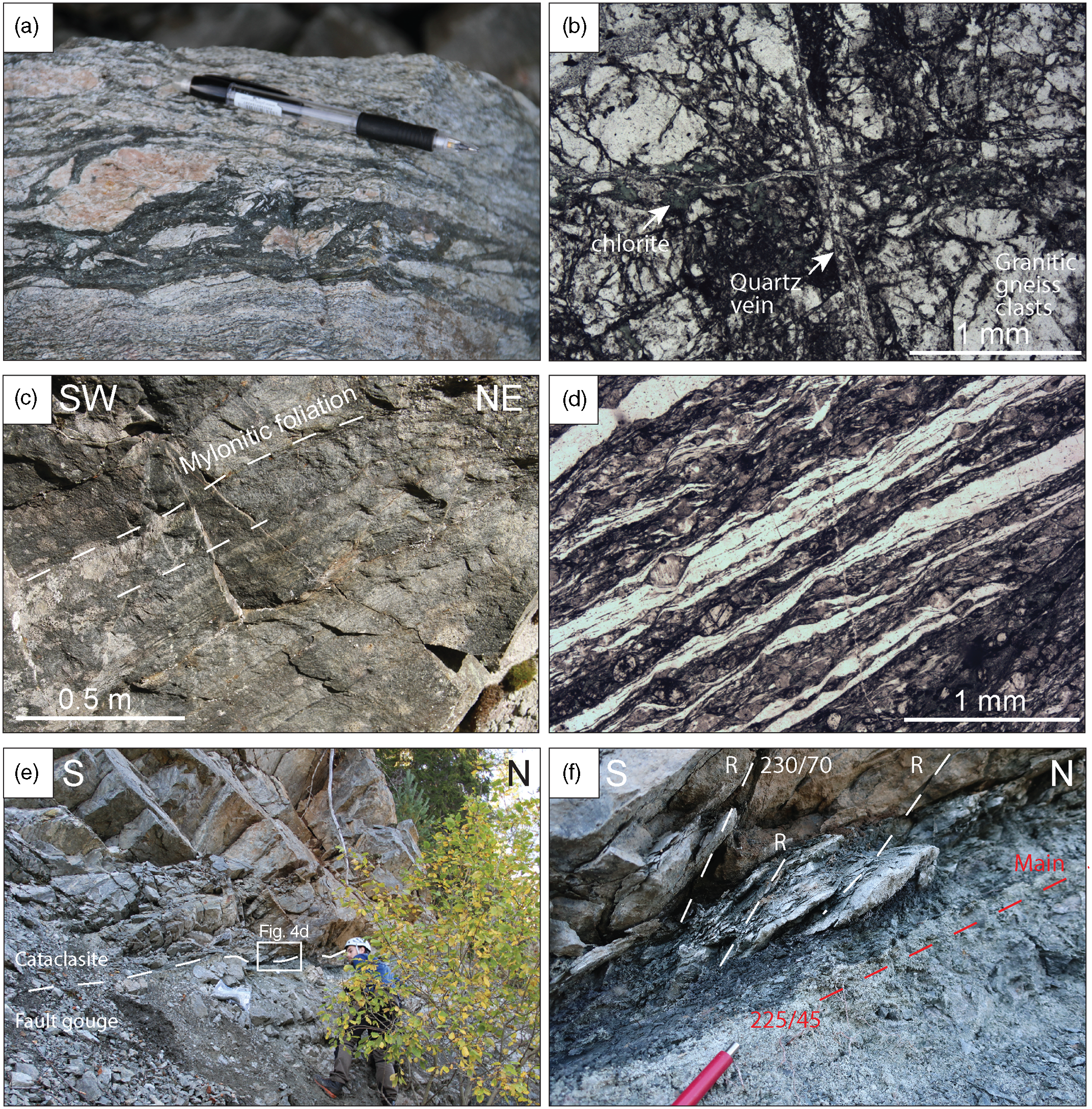
Fig. 4. Field, hand-specimen and thin-section photos of typical deformation rocks in the HØDZ at profile 2 (UTM33N 6632564 293914). (a, b) Cataclasitic band in granitic mylonite in a loose block right next to the cliff (sample RG-16-116). (b) Photomicrograph (plane polarized) of cataclastic granite in (a). (c, d) Amphibolitic mylonite (sample RG16-101), representing the mylonitic core of the HØDZ. Mylonitic foliation (dipdir/dip): 253/62, stretching lineation: 60→222). (d) Photomicrograph (plane polarized) of amphibolitic mylonite in (c). (e) Overview of the contact between fault gouge and overlaying cataclasite. (f) Contact between fault gouge and cataclasite comprises many high-angle secondary fractures interpreted as syntetic riedel shears.
In profile 2, the deepest exposed unit is a protomylonitic granitic gneiss with dark mm-thick zones of ultramylonite or ultracataclasite overprinted by a network of open brittle fractures representing either a lens of deformed country rock or the footwall (Fig. 4a, b). The first interpretation is considered the most likely. In places, the lowermost unit comprises amphibolitic mylonite with microscale asymmetric porphyroclasts (Fig. 4c, d). The protomylonitic granitic gneiss is overlain by a zone of fault gouge / unconsolidated breccia similar to that seen in the lower part of profile 1.
The fault gouge / unconsolidated breccia comprises various subunits described in detail in Section 4.a, below, and has a sharp contact to a c. 0.5 m thick cataclasite above it. The contact is characterized by high-angle fractures interpreted as synthetic riedel shears indicating top-to-the-SW normal displacement (Fig. 4e, f). Profile 2 also contains a deformed dark rock (probably a sheared basic sill) surrounded by recrystallized fine-grained cataclasite/ultracataclasite. The available part of profile 2 is topped by a c. 2 m thick unit of recrystallized protomylonite/blastomylonite.
3.a. Description of geochronological samples
Three fault gouge samples were collected from the two profiles for K–Ar fault gouge geochronology (Figs 1c, 2). At profile 2, a >30 cm thick fault core with two distinct fault gouges is observed (Figs 4e, f, 5a). The lower gouge (BITE133) is at least 15 cm thick, has a sandy to clayey texture and contains abundant angular clasts of the underlying protomylonitic granitic gneiss. The upper gouge (BITE134) is 5–10 cm thick, black to dark grey, without significant visible rock fragments, and with a strongly clayey texture (high plasticity). The contact between the two gouges is sharp and irregular (Fig. 5a). Samples BITE133 and BITE134 were collected c. 10 cm structurally from each other.

Fig. 5. Geochronological samples. (a) Sampling site of fault gouge samples BITE133 and BITE134 showing the textural and structural relationship between the two gouges (profile 2). (b, c) Samples for 40Ar–39Ar dating (B: SB009, C: SB006A). Dated material has been extracted from the grey polygons. K-fsp = K-feldspar, PV = pseudotachylite vein. (d, e) Photomicrograph (crossed polarizers) of SB009 showing dominant euhedral microcrystallite grains with patches of cryptocrystalline and/or amorphous material, and partial melting textures and embayment margins.
Sample BITE135 comes from profile 1 c. 500 m along-strike to the SE from profile 2 (Figs 1c, 2, 3a). The sample is a varicoloured (grey–green to black–brownish), clay-rich sandy gouge that makes up a c. 10 cm thick fault core. The core is situated along the hanging-wall side of a 1.5–2 m wide fault zone composed of highly fractured to brecciated mylonite. The sandy character of the fault gouge is comparable to the grey gouge sampled by BITE133 in profile 2.
Two samples (SB006A and SB009) of protomylonite with strong brittle overprint were selected for 40Ar–39Ar geochronology to date the timing for both visco-plastic and brittle deformation. The samples were collected from profile 1 in a protomylonitic interval immediately above the fault gouge sampled by BITE135 (Figs 1, 2, 5b, c). Both are protomylonitic granitic gneiss with faint (SB009) to moderately (SB006A) developed mylonitic foliation and numerous cross-cutting dark veins (Fig. 5b, c). In SB009, the veins are distinctly black with few protoclasts and sharp, curvilinear boundaries, whereas in SB006A veins are moderate to dark grey, contain abundant protoclasts and commonly have more diffuse, wavy boundaries. SB009 also shows several thin black veinlets emerging from the thicker ones, possibly representing offshoot/injection veins (Fig. 5b). The dark grey veins in SB006A are cut and in places displaced by later quartz veins (Fig. 5c). Thin-section investigation of veins in sample SB009 (Fig. 5d, e) reveals dominant euhedral microcrystallite grains with small patches of cryptocrystalline and/or amorphous material. Partial melting textures and embayment margins are observed and point to conditions of rapid heating and cooling. There are some clasts with sharp edges and minor patches of fibrous, high interference-coloured minerals interpreted as secondary micas (e.g. sericite, chlorite) from devitrification and alteration of the original pseudotachylite (Fig. 5d, e). Near the fine-grained pseudotachylite we observe quartz, plagioclase and lesser amounts of K-feldspar and white micas. Quartz shows dominant crystal–plastic deformation, whereas feldspars are commonly cataclastically deformed. Veins in sample SB006A show some textures that are similar to those in SB009, but are more altered and deformed, indicating significant cataclastic deformation.
Hand specimen and microtextures suggest that the sampled veins represent pseudotachylites that are variably altered and deformed (Kirkpatrick & Rowe, Reference Kirkpatrick and Rowe2013). In the remaining text we will refer to these veins as pseudotachylites, although at least some of the veins also experience significant cataclastic reworking, and a few may be of exclusively cataclastic origin (see discussion in Section 7.b).
4. Methods
4.a. Fault gouge sample preparation
Fault gouge samples were immersed in deionized H2O (15 MΩ) and subjected to c. 100 cycles of freezing and thawing to gently disaggregate clay particles without mechanical grain-size reduction. The samples were subsequently suspended and separated into <2 μm, 2–6 μm and 6–10 μm size fractions by sinking using Stokes’s law. The <2 μm fractions were separated into <0.1 μm, 0.1–0.4 μm and 0.4–2 μm fractions using a Beckman Coulter JCF-Z continuous flow centrifuge. The resulting particle size fractions were concentrated using a high-speed fixed angle rotor, and dried by freeze drying. Dried clay fractions were homogenized using an agate mortar and pestle.
4.b. X-ray diffraction
The mineralogical composition of the different grain-size fractions was studied with X-ray diffraction (XRD) using a Bruker D8 Advance diffractometer with Cu Kα radiation (40 kV / 40 mA) and a Lynxeye XE detector at the Geological Survey of Norway, Trondheim. XRD scans were carried out for 3–75° 2θ and a step size of 0.02°. Signal acquisition time was 1 s per step. The optical system was equipped with soller slits (2.5°) and a fixed divergence slit (0.6 mm). Randomly oriented specimens were run for subsequent Rietveld modelling. For accurate clay mineral identification, oriented specimens on glass slides were measured at room temperature, after glycolization for 24 hours and after heat treatment for 1 hour at 550 °C. Mineral identification was carried out with Bruker’s Diffrac.EVA 4.2 using the International Centre for Diffraction Data (ICDD)’s PDF4 Minerals as well as the Crystallographic Open Database. Quantification was performed with TOPAS 5, and refined parameters included unit cell dimensions, sample displacement, preferred orientation as well as background coefficients. Limit of quantification and uncertainty is mineral-dependent, but commonly 1–2 wt % and 2–3 wt %, respectively. Illite crystallinity (Kübler Index (KICIS)) was determined from the full width at half-maximum (FWHM) of the 10 Å peak and CIS-standardized according to Warr (Reference Warr2018) and Warr & Rice (Reference Warr and Rice1994).
4.c. K–Ar analysis
Splits of air-dried, homogenized clay materials and standards were packed in weighed molybdenum envelopes, and the net mass of the aliquots was determined using a Mettler Toledo XPE26DR microbalance fitted with an antistatic ionizer. The microbalance has a resolution of 2 µg and a measured reproducibility of 4 µg (1σ). The clays and standards were left overnight in a drying oven at 85 ± 3 °C, and then left to cool in an exicator. The molybdenum envelopes were subsequently loaded into a stainless-steel ultra-high-vacuum extraction line, and baked at a maximum temperature of 120 °C to eliminate excess water, while avoiding unwanted 40Ar* loss from the samples, following the recommendations of Clauer & Chaudhuri (Reference Clauer and Chaudhuri1995).
Argon was extracted from the aliquots for 20 min at 1400 °C in a Pond Engineering double vacuum resistance furnace. During heating, bulk sample gas was expanded directly into a stainless-steel vessel housing a freshly activated Titanium Sublimation Pump, to strip the sample gas from a majority of reactive gases including H2O, N, O, CO and CO2 (O’Hanlon, Reference O’Hanlon2005). Purified sample gas was spiked with a known amount (c. 2 × 10−13 moles) of pure 38Ar spike (Schumacher, Reference Schumacher1975) and equilibrated for 2 min. The gas mixture was subsequently isolated in a second clean-up stage and exposed for 10 min to two SAES GP50 getter cartridges with ST101 Zr–Al alloy, one of which was kept at 350 °C and one at room temperature, to remove residual reactive gases including H2 and CH4.
Argon isotopes were determined on an IsotopX NGX multicollector noble gas mass spectrometer using faraday cups fitted with 1012 Ω amplifiers, except for 40Ar which was measured using a faraday fitted with a 1011 Ω amplifier. Time-zero beam intensities were measured for 30 cycles of 20 1-s integrations, and time-zero intensities were calculated using exponential regressions back to gas inlet time. Furnace blanks were run regularly between samples, and had Ar compositions comparable to atmospheric argon. Instrument mass discrimination was determined within this analytical batch by a comparing a weighted mean of 50 analyses of atmospheric argon (40Ar/36Ar = 299.579 ± 0.012) with the reference value of 298.56 ± 0.31 (Lee et al. Reference Lee, Marti, Severinghaus, Kawamura, Yoo, Lee and Kim2006). The 38Ar spike pipette was calibrated using GA-1550 biotite with 40Ar* = 1.342 ± 0.007 × 10−9 mol g−1 (McDougall & Wellman, Reference McDougall and Wellman2011) and HD-B1 biotite (Fuhrmann et al. Reference Fuhrmann, Lippolt and Hess1987) with a 40Ar* = 3.351 ± 0.01 × 10−10 mol g−1 (Charbit et al. Reference Charbit, Guillou and Turpin1998). The overall standard deviation of the pooled spike calibrations by combined GA1550 and HD-B1 is <0.3 %. The accuracy of the 40Ar* determinations was monitored within-run by HD-B1 biotite.
Potassium concentration was determined by digesting a sample aliquot of ∼50 mg of sample material in Li2B4O7 flux at a temperature of 1000 ± 50 °C in palladium crucibles. The resulting glass was subsequently dissolved in HNO3, and analysed on an Agilent 5110 VDV ICP-OES. 1σ uncertainties depend on the sample weight and its K concentration, and are typically 1.5 % relative for pure mica, as determined by repeated measurements of geological standards DNC dolerite, Mica-Mg phlogopite and MA-N granite.
K–Ar ages were calculated using the 40K decay constants, abundance and branching ratio of Steiger & Jäger (Reference Steiger and Jäger1977). Atmospheric argon corrections were performed using the relative abundances of 40Ar, 38Ar and 36Ar of Lee et al. (Reference Lee, Marti, Severinghaus, Kawamura, Yoo, Lee and Kim2006; 40Ar/36Ar = 298.56 ± 0.31). 1σ uncertainties were estimated using the error equation for multicollector isotope dilution measurements from Hałas & Wójtowicz (Reference Hałas and Wójtowicz2014) modified to take into account the uncertainty on mass discrimination.
4.d. 40Ar–39Ar analysis
The samples were cut into slabs, c. 0.3–0.5 cm thick. A micro rotary tool was then used under flowing water to cut ∼0.2 cm3 chips from the pseudotachylites (see Fig. 5b, c for sampling sites). The vein chips were further crushed with a mortar. Care was taken not to include larger host rock fragment entrained in the melt matrix; however, this possibility cannot be ruled out. In particular, the pseudotachylite in sample SB006A was less defined (thinner) and harder to separate from the host rock fragments (Fig. 5c). From the same rock slabs, host rock micas and feldspars were separated applying normal methods. The analytical protocol follows Vissers et al. (Reference Vissers, Ganerød, Pennock and van Hinsbergen2020). The raw blank-corrected mass spectrometer outputs can be found in Table S1 in the Supplementary Material available online at https://doi.org/10.1017/S0016756822000966, and the main results in Table 3 (further below).
5. Results
5.a. Fault gouge mineralogy
All three fault gouge samples from the Himdalen Fault contain dominant illite/muscovite, chlorite and smectite (Table 1; XRD spectra in Fig. S2 in the Supplementary Material available online at https://doi.org/10.1017/S0016756822000966). Although small amounts of K-feldspar are present in the three coarsest fractions of BITE134 and BITE135, illite/muscovite is the only K-bearing phase in the two finest size fractions of all three samples. Quartz is present in moderate to minor amounts in the coarser fractions of all three samples, whereas significant concentrations of plagioclase are only present in BITE133. The difference in content of wall-rock derived plagioclase between BITE133 and BITE134 is consistent with the observed contrast in fault gouge characteristics, wherein BITE134 is significantly finer-grained than BITE133 and contains no observable wall rock fragments. Minor to trace amounts of K-feldspar are present in the coarsest fractions of BITE134 and BITE135.
Table 1. XRD results (wt %), including illite crystallinity (Kübler Index KICIS)

Notes: Mineral abbreviations: ill/musc, illite/muscovite; K-fsp, alkali-feldspar; chl, chlorite; sm, smectite; qtz, quartz; plag, plagioclase; ant, anatase; cal, calcite; rt, rutile; GOF, goodness of fit; Rwp, weighted profile factor. Both GOF and Rwp represent accuracy parameters for mineral quantification by Rietveld modelling.
* Concentrations are only approximate, due to poor sample crystallinity. Smectite concentration is most likely underestimated.
The crystallinity of illite varies significantly within each sample from anchizone (KICIS of 0.36) to early diagenetic (KICIS of 1.85; Table 1). The KI is strongly positively correlated with grain size, where illite in the coarser size fractions has higher crystallinity than in the finer size fractions. Notably, illite in the coarsest size fractions of samples BITE133 and BITE134 has significantly different KI values (KICIS = 0.88–1.08 vs 0.36–0.64, respectively; Table 1), although the samples were collected only 10 cm from each other. The KI values of the coarsest fractions in BITE135 are comparable to those in BITE133.
Qualitative assessment of illite polytypes (2M1 and 1M-1Md; Velde, Reference Velde1965) shows that all size fractions are dominated by poorly crystalline 1M illite. Size fraction 0.1–0.4 µm in BITE135 also contains minor amounts of smectitic interlayers in the illite. Well-ordered 2M1 illite is subordinate in the coarsest fraction (6–10 µm) of BITE135, as evidenced by a minor peak at 2.8 Å (Fig. S2 in the Supplementary Material available online at https://doi.org/10.1017/S0016756822000966; Grathoff & Moore, Reference Grathoff and Moore1996). The predominance of low-temperature, disordered 1M illite is consistent with anchizone- to early diagenetic-grade illite crystallinities (Table 1). The 1M illite likely formed by dissolution of wall-rock-derived K-feldspar (Haines & van der Pluijm, Reference Haines and van der Pluijm2012).
5.b. K–Ar fault gouge ages
A total of 15 K–Ar ages were obtained from the three Himdalen Fault gouge samples (Table 2). All fractions have moderate K concentrations (from 1.192 to 3.583 wt %) and the Ar is dominantly radiogenic (49.5 to 92.7 %). The ages vary from 387.2 ± 6.0 Ma to 195.8 ± 6.3 Ma, and all samples show consistently decreasing ages with grain size. The finest size fractions (<0.1 µm) of all samples have nearly identical ages (from 195.8 ± 3.2 to 205.0 ± 3.5 Ma), and the coarsest fractions for BITE133 and BITE135 overlap within error (380.6 ± 6.6 and 387.2 ± 6.0 Ma). The ages of the three coarsest fractions both in BITE134 and BITE133 are identical within error at c. 268 Ma and c. 375 Ma, respectively.
Table 2. K–Ar fault gouge results

5.c. 40Ar–39Ar ages
White mica and K-feldspar from sample SB006A (host rock) defines overlapping age steps of parts of the degassing spectrum (Fig. 6a) and yielded inverse variance weighted means of 908.6 ± 7.0 Ma and 435.4 ± 23.8 Ma, respectively (Table 3). The degassing spectrum from the pseudotachylite of this sample (Fig. 6b) shows two mini-plateaus of 375.0 ± 22.7 Ma and 639.5 ± 18.8 Ma, respectively.
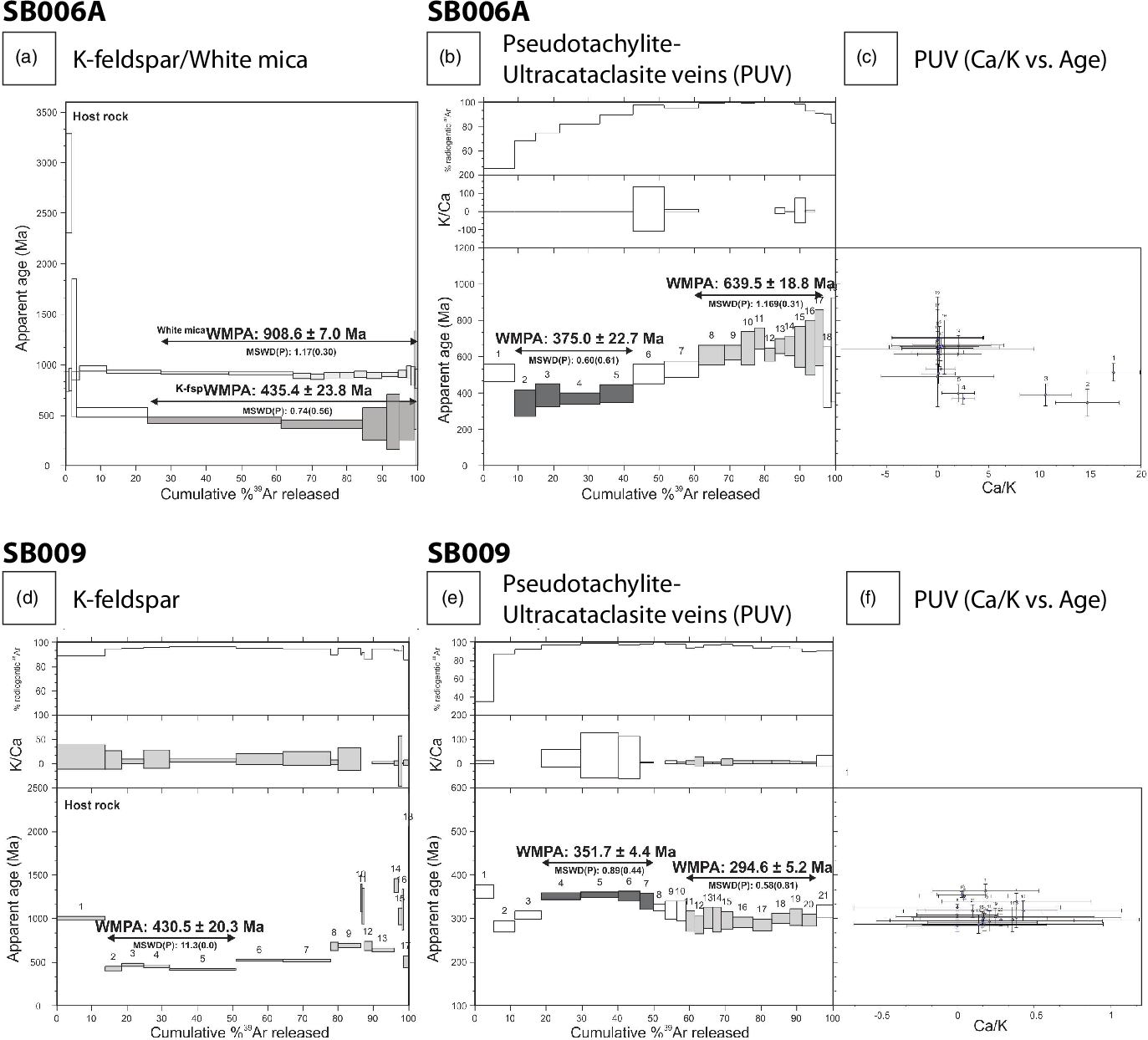
Fig. 6. 40Ar–39Ar degassing spectra (a, b, d, e), with Ca/K vs age plots (c and f) for the pseudotachylite vein analyses.
Table 3. 40Ar–39Ar results

Host rock K-feldspar from sample SB009 reveals a disrupted age pattern (Fig. 6d); however, steps 2–5 hint at a resemblance to samples SB006A (430.5 ± 20.3 Ma; Fig. 6b), although the statistical basis for this is poor. The pseudotachylite samples reveal two mini-plateaus, one at 351.7 ± 4.4 Ma and the other at 294.6 ± 5.2 Ma at higher-temperature steps (Fig. 6e).
In both pseudotachylite samples, there are distinct younger age populations compared to the host rock samples. Both carry a late Devonian signal (375.0 ± 22.7 Ma and 351.7 ± 4.4 Ma), both of which are statistically within error. However, they behave differently at higher-temperature steps. Sample SB006A climbs to c. 640 Ma, whereas SB009 defines a clear plateau at c. 295 Ma. We speculate whether this up-climbing pattern in sample SB006A could be due to the presence of a higher portion (mix) of unmelted material from the source rock with different diffusive properties as seen in many other natural pseudotachylites (e.g. Di Vincenzo et al. Reference Di Vincenzo, Rocchi, Rossetti and Storti2004). The Ca/K data (calculated from 37Ar–39Ark; Fig. 6c) indicate at least two Ar reservoirs, from high Ca/K ratios (e.g. plagioclase) to low ratios. Sample SB009 drops down to a plateau of 294.6 ± 5.2 Ma (Fig. 6e). Judging from the Ca/K data for this sample, the gas comes from high-K reservoirs, probably host-rock K-feldspar (351.7 ± 4.4 Ma) and a melt-matrix-dominated reservoir (294.6 ± 5.2 Ma).
6. Discussion
The presented structural and geochronological results show that the HØDZ is a multi-reactivated deformation zone where repeated brittle deformation events have exploited and partly overprinted an older mylonitic fabric associated with the Ørje Shear Zone. Below we first interpret the K–Ar geochronological dataset, before discussing the regional implications of the new deformation ages. A brief discussion of brittle localization in visco-plastic shear zones follows at the end.
6.a. Interpreting K–Ar geochronological data of a multi-reactivated fault
K–Ar fault gouge dating is not a straightforward geochronological method but requires careful analyses of each individual sample through integration of the isotope data with other constraints, such as field and microscopic observations, mineralogical quantification and external geochronometers and geothermometers (van der Pluijm et al. Reference van der Pluijm, Hall, Vrolijk, Pevear and Covey2001; Zwingmann & Mancktelow, Reference Zwingmann and Mancktelow2004; Haines & van der Pluijm, Reference Haines and van der Pluijm2008; Viola et al. Reference Viola, Scheiber, Fredin, Zwingmann, Margreth and Knies2016; Vrolijk et al. Reference Vrolijk, Pevear, Covey and LaRiviere2018; Scheiber et al. Reference Scheiber, Viola, van der Lelij, Margreth and Schönenberger2019; Mottram et al. Reference Mottram, Kellett, Barresi, Zwingmann, Friend, Todd and Percival2020; Tartaglia et al. Reference Tartaglia, Viola, van der Lelij, Scheiber, Ceccato and Schönenberger2020; Hueck et al. Reference Hueck, Wemmer, Ksienzyk, Kuehn and Vogel2022; Viola et al. Reference Viola, Musumeci, Mazzarini, Tavazzani, Torgersen, van der Lelij and Aldega2022). Below we discuss why we interpret the HØDZ fault gouge geochronological results to constrain faulting at c. 380, c. 270 and c. 200 Ma.
The most robust constraint on the absolute timing of fault activity along the Himdalen Fault is represented by the ages of the finest size fraction (<0.1 µm) in all three gouge samples, which are nearly identical within error at 196.5 ± 6.3 Ma, 205.0 ± 3.5 Ma and 195.8 ± 3.2 Ma (BITE133, 134 and 135, respectively; Table 2; Fig. 7). The obtained age of the finest fraction (<0.1 µm) is commonly interpreted as the age of the last datable increment of faulting, as this fraction contains dominantly authigenic syn-kinematic illite (e.g. Zwingmann et al. Reference Zwingmann, Offler, Wilson and Cox2004; Wemmer et al. Reference Wemmer, Steenken, Müller, de Luchi and Siegesmund2011; Ksienzyk et al. Reference Ksienzyk, Wemmer, Jacobs, Fossen, Schomberg, Süssenberger, Lünsdorf and Bastesen2016). The finest fraction may contain traces of inherited grains, but only in very rare cases do these contribute significantly to the obtained K–Ar age (e.g. Viola et al. Reference Viola, Torgersen, Mazzarini, Musumeci, van der Lelij, Schönenberger and Garofalo2018). Typically, the most common contaminant in the finest size fraction is wall-rock-derived 2M1 illite/muscovite. Its effect on the obtained age may be corrected for by the Illite Age Analysis approach (IAA; Pevear, Reference Pevear1999; van der Pluijm et al. Reference van der Pluijm, Hall, Vrolijk, Pevear and Covey2001). However, the samples presented in this study almost exclusively comprise poorly crystalline, authigenic 1M illite (Table 1; Fig. S2 in the Supplementary Material available online at https://doi.org/10.1017/S0016756822000966), which precludes the use of IAA. The fact that all three samples converge to a similar age for their finest size fraction is a strong argument for interpreting this age (c. 200 Ma) as geologically meaningful. Conservatively, this age should be regarded as a maximum age of the last period of fault slip (e.g. Zwingmann & Mancktelow, Reference Zwingmann and Mancktelow2004; Tartaglia et al. Reference Tartaglia, Viola, van der Lelij, Scheiber, Ceccato and Schönenberger2020).

Fig. 7. Compilation of K–Ar and XRD analyses of the fault gouges. (a) Age vs grain-size plot. Solid marker: interpreted as geologically significant; transparent marker: interpreted as a mixed age and therefore geologically insignificant. (b) Age vs illite crystallinity (Δ 2°θ). Yellow horizontal bars indicate the interpreted faulting events recorded by the fault gouge samples. (c) Mineralogy of the dated samples. Notes on XRD-columns: <<0.1 µm fractions in samples BITE133 and BITE135 do not yield enough material for XRD analysis. * Analysed in low-background Si-sample holders with depressions. **Concentrations are only approximate due to poor sample crystallinity. Smectite concentration is most likely underestimated.
The c. 200 Ma faulting event is not the only activity recorded by the Himdalen Fault. The field relationships disclose a multiphase brittle history with a distinct difference in character between the grey, fragment-rich gouges (BITE133 and 135) and the black, fine-grained gouge (BITE134; Fig. 5a). The latter appears more deformed and contains much more illite than the former. The apparent overprinting relationship of the black gouge at the expense of the grey at profile 2 (Figs. 2, 5a) suggests that the black gouge partially reworked the former by continued crushing and mineral transformations. Similar situations of partial reactivation and overprinting of fault gouges have been documented in several other fault zones (Viola et al. Reference Viola, Zwingmann, Mattila and Kapyaho2013, Reference Viola, Musumeci, Mazzarini, Tavazzani, Torgersen, van der Lelij and Aldega2022; Torgersen et al. Reference Torgersen, Viola, Zwingmann and Harris2015 a; Tartaglia et al. Reference Tartaglia, Viola, van der Lelij, Scheiber, Ceccato and Schönenberger2020). This first-order relative age difference is supported by the presence of significant amounts of wall-rock-derived minerals (e.g. plagioclase) in the three coarsest fractions of BITE133, but not in the overprinting BITE134 (Table 2; Fig. 7).
Although the three fault gouges show a common age for the finest fraction, reflecting that they all experienced significant new illite growth during the latest datable reactivation event, the timing of their initial development differs. The observed relative age difference between BITE133 and BITE134 is recorded by the K–Ar ages of the three coarsest fractions in both samples: the black gouge (BITE134) is about 100–110 Ma younger than the neighbouring grey gouge (BITE133). BITE134, in turn, overlaps with the 6–10 µm fraction age of BITE135 (Table 2; Fig. 7). Age plateaus in K–Ar age-versus-grain-size space, termed pseudo-plateau ages, are defined by two or more neighbouring size fractions with the same age and are observed in many fault-dating studies (Torgersen et al. Reference Torgersen, Viola, Zwingmann and Harris2015 a; Aldega et al. Reference Aldega, Viola, Casas-Sainz, Marcén, Román-Berdiel and van der Lelij2019; Scheiber et al. Reference Scheiber, Viola, van der Lelij, Margreth and Schönenberger2019). Pseudo-plateau ages indicate that the fault gouge contains a significant population of mineral grains with similar age, and therefore pseudo-plateau ages can be interpreted as robust and geologically significant (Scheiber et al. Reference Scheiber, Viola, van der Lelij, Margreth and Schönenberger2019).
It has previously been proposed that the coarsest fractions of fault gouges consist predominantly of protolithic minerals inherited from the wall rocks (e.g. van der Pluijm et al. Reference van der Pluijm, Hall, Vrolijk, Pevear and Covey2001). However, several recent studies show that in multi-reactivated fault gouges, the coarsest fractions may be dominated by authigenic, syn-kinematic minerals (herein called proto-authigenic minerals) that are associated with an earlier faulting phase than that recorded by the finest size fraction (Torgersen et al. Reference Torgersen, Viola, Zwingmann and Harris2015 a; Ksienzyk et al. Reference Ksienzyk, Wemmer, Jacobs, Fossen, Schomberg, Süssenberger, Lünsdorf and Bastesen2016; Viola et al. Reference Viola, Scheiber, Fredin, Zwingmann, Margreth and Knies2016; Kemp et al. Reference Kemp, Gillespie, Leslie, Zwingmann and Campbell2019). The obtained ages of the coarsest fractions should therefore be carefully evaluated with regard to the dominance of either protolithic or proto-authigenic minerals, or a mixture between them. To do so, several factors must be considered, including (a) which K-bearing mineral phases are present, (b) the amount of each mineral, (c) their K-content, (d) whether they are wall-rock-derived or authigenic, (e) their theoretical K–Ar closure temperature and (f) the approximate temperatures during faulting.
Illite/muscovite is the dominant contributor to the obtained K–Ar ages of the coarsest fractions in all three samples (Table 1). If the illite/muscovite was well-ordered 2M1 muscovite derived from the host mylonites (i.e. protolithic grains), the age should approach that constrained by the 40Ar–39Ar date of 908.6 ± 7.0 Ma (Table 3; Fig. 6). However, the samples contain no 2M1 illite/muscovite and the anchizone to diagenetic zone KI values (Table 1; Fig. S2 in the Supplementary Material available online at https://doi.org/10.1017/S0016756822000966; Fig. 7) strongly suggest that the illite/muscovite is authigenic. We therefore interpret the pseudo-plateau ages of BITE133 and BITE134 (c. 375 Ma and 268 Ma, respectively) to date syn-kinematic, authigenic illite/muscovite formed during two significant faulting episodes. The difference in age correlates with a shift in illite crystallinity between the black gouge fractions (KICIS = 0.88–1.08) and those from the grey gouges (KICIS = 0.36–0.64; Table 1; Fig. 7). This shift indicates that the fault reactivation event leading to the development of the black gouge likely took place at lower temperatures than the faulting episode causing the formation of the grey gouges. At the same time, this is significantly higher than that of the younger (c. 200 Ma) finest fraction (KICIS = 1.85).
It should be noted here that it is a very common phenomenon that the Kübler Index, i.e. the FWHM of the (001) illite peak, increases with decreasing grain size. This means that the highest KI values are recorded for the <0.1 µm fraction and often they are automatically assigned diagenetic formation conditions (Schomberg et al. Reference Schomberg, Wemmer, Warr and Grathoff2019). At such small grain sizes, however, peak broadening is frequently observed and results from small crystallite sizes. The question arises whether formation conditions can be interpreted from the KI values of the finest size fractions (<<2 µm), or if increased KI values for these size fractions are solely because the grains are smaller. Independent temperature (and pressure) constraints such as fluid inclusion analyses would be very useful in future studies to confirm the assumptions derived from KI values.
The pseudo-plateau ages may be affected by the presence of K-feldspar and/or plagioclase, which are present in variable amounts in all three samples. BITE134 and BITE135 contain <5 wt % and <13 wt %, respectively, of mainly K-feldspar (Table 1). 40Ar–39Ar dating of K-feldspar from the host rock shows that these have significantly older Ar ages than the fault gouges (435.4 ± 23.8 Ma and 430.2 ± 20.3 Ma; Table 3; Fig. 6), suggesting that either (1) their effect on the obtained K–Ar ages is insignificant or (2) they crystallized or were isotopically reset at the same time as the illite. BITE133 contains noteworthy amounts of plagioclase (17–48 wt %; Table 1). Although plagioclase usually contains only trace amounts of K it may comprise up to 1 % K (e.g. Heier, Reference Heier1962), which means that contribution of plagioclase to the age cannot be completely ruled out without further analysis. However, given that the three coarsest fractions of BITE133 overlap in age despite a large variation in plagioclase content (17–48 wt %), and that its pseudo-plateau age overlaps with the age of the coarsest fraction in BITE135 (Fig. 7), as well as with the 40Ar–39Ar plateau age of pseudotachylite in sample SB006A (Fig. 6), it is very unlikely that plagioclase contributes significantly to the age of these grain-size fractions. We therefore interpret the pseudo-plateau ages in the HØDZ fault gouges to represent significant faulting events at c. 380 Ma and c. 270 Ma (Fig. 7).
6.b. Interpretation of 40Ar–39Ar pseudotachylite ages
The dated pseudotachylites show two-plateau degassing spectra with similar ages for the low-temperature steps (375.0 ± 22.7 Ma and 351.7 ± 4.4 Ma), but different ages for the high-temperature steps (294.6 ± 5.2 Ma and 639.5 ± 18.8 Ma; Fig. 6; Table 3). Most of the pseudotachylite plateau ages are significantly younger than those in the host rock, which matches well the cross-cutting nature of the pseudotachylites (Fig. 5b, c) and strongly indicates that these plateau ages date deformation rather than regional cooling.
One of the plateau ages, at c. 640 Ma (SB006A), is older than K-feldspar in the host mylonites (c. 435 Ma) and, thus, likely to represent an inherited component or mix of components, possibly also partially reset. Such an interpretation is consistent with the presence of significant protoclasts in the SB006A pseudotachylites (Fig. 5b, c). SB009, which do not have a c. 640 Ma age signal, also contains significantly fewer protoclasts. An alternative interpretation is that the c. 640 Ma plateau age dates cataclastic deformation of the mylonite that was in turn reworked by the pseudotachylites. Such an interpretation is consistent with the widespread cataclastic deformation textures both at outcrop- and microscale but requires that the dated vein material has a higher closure temperature than K-feldspars in the mylonites. Such material could possibly be syn-deformational crystallized micas and/or partially melted micas.
The three remaining plateaus constrain, within error, two age groups (380–350 Ma and c. 295 Ma). Based on field observation, microscale textures and geochronological data, we suggest that the two ages are of different origin – pseudotachylite and cataclasite.
The main argument in favour of such an interpretation comes from the age spectrum of SB009. Here the high-temperature steps (11–20) constrain a younger plateau age (c. 295 Ma) than the lower-temperature plateau (4–7; c. 350 Ma; Fig. 5e), which suggests that the c. 295 Ma signal originates from a more retentive source than the c. 350 Ma signal. We interpret the two sources to be melt-dominated and cataclastic-dominated matrix, respectively, which is consistent with hand specimen and microscale textures and structures typical for both pseudotachylite and cataclasite (Fig. 5b–e). The existence of an episode of significant cataclastic deformation pre-dating pseudotachylite formation is also supported by the fact that the plateau ages of SB009 and SB006A overlap internally (Fig. 6b, e), as well as with the K–Ar ages of the coarsest size fractions of the two fragment-rich fault gouges (BITE133 and 135; Fig. 7).
Sherlock et al. (Reference Sherlock, Strachan and Jones2009) suggest that cataclasites are unsuitable for argon dating because they are only mechanically crushed and therefore not outgassed. However, recent studies have shown that cataclasitic deformation may comprise considerable authigenic mineral growth (e.g. Schleicher et al. Reference Schleicher, Sutherland, Townend, Toy and van der Pluijm2015; Gundlach-Graham et al. Reference Gundlach-Graham, Garofalo, Schwarz, Redi and Günther2018) and cause considerably increased Ar diffusivity, which may lead to complete resetting of the Ar system (e.g. in K-feldspar; Wang et al. Reference Wang, Zhou, Zwingmann, Lo, Li and Hao2020). In the case of the HØDZ, feldspars in and around the pseudotachylites show significant fracturing and cataclastic deformation (Fig. 5d, e). Dissolution of fractured feldspar may lead to precipitation of poorly crystalline 1M illite (Haines & van der Pluijm, Reference Haines and van der Pluijm2012). Indeed, authigenic mineral growth is documented in the HØDZ by the predominance of 1M illite in the coarse fault gouge fractions with ages around 380 Ma (Table 1; Fig. S2 in the Supplementary Material available online at https://doi.org/10.1017/S0016756822000966).
In summary, the pseudotachylite age spectra are complex, and a unique interpretation is not possible from the presented data alone. The most consistent and plausible interpretation is that the 40Ar–39Ar plateau ages date early cataclastic deformation around 380–350 Ma and pseudotachylite formation at 294.6 ± 5.2 Ma. Field, hand specimen and microscale observations demonstrate widely distributed cataclastic deformation that is cut by pseudotachylite veins (Figs. 4a, b, 5b, c). The interpretation implies that only SB009 pseudotachylite preserves enough material to resolve a c. 295 Ma frictional melting–cooling event.
6.c. Regional implication of the new deformation ages
The HØDZ has accommodated multiple deformation events at different conditions, separated by millions of years of inactivity. It therefore provides a 700 Ma archive of crustal deformation in southeast Norway, which is discussed below and summarized in Figure 7.
6.c.1. Early Neoproterozoic greenschist facies extensional reactivation (Fig. 8a)

Fig. 8. Graphic summary of the recorded deformation events along the Himdalen–Ørje Deformation Zone and its interpreted regional context. Age data in regional events maps from 1. Mulch et al. (Reference Mulch, Cosca, Andresen and Fiebig2005); 2. Viola et al. (Reference Viola, Henderson, Bingen and Hendriks2011); 3. Tillberg et al. (Reference Tillberg, Drake, Zack, Kooijman, Whitehouse and Åström2020); 4. Viola et al. (Reference Viola, Scheiber, Fredin, Zwingmann, Margreth and Knies2016), Scheiber & Viola (Reference Scheiber and Viola2018) and Scheiber et al. (Reference Scheiber, Viola, van der Lelij, Margreth and Schönenberger2019); 5. Ksienzyk et al. (Reference Ksienzyk, Wemmer, Jacobs, Fossen, Schomberg, Süssenberger, Lünsdorf and Bastesen2016); 6. Eide et al. (Reference Eide, Torsvik, Andersen and Arnaud1999); 7. Torgersen et al. (Reference Torgersen, Viola, Zwingmann and Henderson2015 b); 8. Fossen et al. (Reference Fossen, Ksienzyk, Rotevatn, Bauck and Wemmer2021); 9. Sherlock et al. (Reference Sherlock, Watts, Holdsworth and Roberts2004); 10. Tartaglia et al. (Reference Tartaglia, Viola, van der Lelij, Scheiber, Ceccato and Schönenberger2020); 11. Ferstad (Reference Ferstad2017); 12. Hestnes et al. (Reference Hestnes, Gasser, Scheiber, Jacobs, van der Lelij, Schönenberger and Ksienzyk2022). Offshore regional events based mostly on Kalani et al. (Reference Kalani, Faleide and Gabrielsen2020). Abbrevations: SDF: Scandinavian Deformation Front; MZ: Mylonite Zone; KPSZ: Kristiansand–Porsgrunn Shear Zone; ST: Sorgenfrei–Tornquist Zone; MTFC: Møre-Trøndelag Fault Complex; NSDZ: Nordfjord–Sogn Detachment Zone; HSZ: Hardangerfjord Shear Zone; RDZ: Røldal Shear Zone; OG: Oslo Graben; OR: Oslo Rift; SG: Skagerrak Graben; LGF: Lærdal Gjende Fault; VG: Viking Graben; CG: Central Graben.
The oldest deformation rocks within the HØDZ are the mylonites making up the Ørje Shear Zone. 40Ar–39Ar dating of white mica from these mylonites gives an age of 908.6 ± 7.0 Ma (Figs 6, 8). This age overlaps with 40Ar–39Ar ages from other large shear zones within the Sveconorwegian Orogen (Fig. 8a), such as the Mylonite Zone (c. 922–860 Ma; Viola et al. Reference Viola, Henderson, Bingen and Hendriks2011) and the Kristiansand–Porsgrunn Shear Zone (c. 890–880 Ma; Mulch et al. Reference Mulch, Cosca, Andresen and Fiebig2005). These deformation ages are invariably interpreted to date late-orogenic extensional reactivation of pre-existing compressional shear zones. We suggest a similar interpretation of the HØDZ white mica age. Given its parallelism to the northern segment of the Mylonite Zone, it likely also accommodated sinistral oblique top-to-the-W slip during this event (Fig. 8a; Viola et al. Reference Viola, Henderson, Bingen and Hendriks2011).
6.c.2. Late Neoproterozoic activity?
During the late Neoproterozoic break-up of Rodinia, major brittle deformation led to the development of multiple rift basins in Baltica (Kumpulainen & Nystuen, Reference Kumpulainen, Nystuen, Gee and Sturt1985). The 40Ar–39Ar plateau age of 639.5 ± 18.8 Ma from one of the pseudotachylites overlaps with the timing of this major rifting event (Pease et al. Reference Pease, Daly, Elming, Kumpulainen, Moczydlowska, Puchkov, Roberts, Saintot and Stephenson2008; Kjøll, Reference Kjøll2020), indicating that the HØDZ may have been active at this time interval. However, the geological significance of the c. 640 Ma age is highly uncertain (as described in Section 7.b. above) and more structural–geochronological analysis of the HØDZ is required to verify its possible late Neoproterozoic deformation history.
6.c.3. Silurian cooling ages associated with the Caledonian orogeny (Fig. 8b)
The HØDZ seems largely unaffected by the Caledonian orogeny (c. 480–400 Ma; Corfu et al. Reference Corfu, Andersen, Gasser, Corfu, Gasser and Chew2014), although the study site sits close to the easternmost extent of the Caledonian front. The only ‘Caledonian’ ages in the HØDZ come from K-feldspar in the mylonites (Table 3; Fig. 6). Although the 40Ar–39Ar plateau ages of both K-feldspar samples have large errors (435.4 ± 23.8 Ma and 430.2 ± 20.3 Ma), they are similar to the stratigraphically constrained Wenlock–Ludlow age (c. 430–420 Ma) of major Caledonian contraction in the Osen – Røa Nappe Complex within the Oslo Graben (Fig. 8b; Bruton et al. Reference Bruton, Gabrielsen and Larsen2010). However, it is considered highly unlikely that the HØDZ accommodated any Caledonian strain as it is oriented nearly parallel to the main Caledonian ESE transport direction in this area. We therefore interpret the c. 435 Ma K-feldspar ages as cooling ages linked to the effects of the advancing and collapsing Caledonian orogen.
6.c.4. Late Devonian – Early Carboniferous brittle reactivation (Fig. 8b)
The most unexpected deformation ages obtained in this study are the Late Devonian to Early Carboniferous 40Ar–39Ar plateau ages of the pseudotachylites and the K–Ar ages of the coarsest fraction of two of the fault gouges (385–350 Ma; Figs. 6–8). There are very few similar metamorphic or deformation ages in southeast Norway and southern Sweden. The only exceptions are a number of slickensided fracture surfaces at Forsmark, eastern Sweden, which give Rb–Sr isochrons between 398.6 ± 5.1 Ma and 348.9 ± 8.6 Ma (Tillberg et al. Reference Tillberg, Drake, Zack, Kooijman, Whitehouse and Åström2020), and a series of metamict zircons in central Sweden with a lower intercept U–Pb discordia age of 384 ± 15 Ma (Fig. 8b; Högdahl et al. Reference Högdahl, Gromet and Broman2001). In Baltica, the major tectonic event at that time was the collapse of the Scandinavian Caledonides. The collapse took place through several stages along dominating NW–SE to E–W extension directions (Osmundsen & Andersen, Reference Osmundsen and Andersen2001; Fossen, Reference Fossen, Law, Butler, Holdsworth, Krabbendam and Strachan2010; Braathen et al. Reference Braathen, Osmundsen, Maher and Ganerød2018; Wiest et al. Reference Wiest, Jacobs, Fossen, Ganerød and Osmundsen2021), and is particularly well recorded by the large extensional detachment zones and supradetachment basins in western Norway (e.g. Osmundsen & Andersen, Reference Osmundsen and Andersen2001). Alkali feldspar 40Ar–39Ar thermochronology points toward a major phase of unroofing in the Early Carboniferous (360–340 Ma; Eide et al. Reference Eide, Torsvik, Andersen and Arnaud1999), and both Viola et al. (Reference Viola, Scheiber, Fredin, Zwingmann, Margreth and Knies2016) and Ksienzyk et al. (Reference Ksienzyk, Wemmer, Jacobs, Fossen, Schomberg, Süssenberger, Lünsdorf and Bastesen2016) present Early Carboniferous (c. 350 Ma) faulting ages for two separate faults in western Norway (Fig. 8b). However, there is no documentation of any post-collisional extensional deformation in the foreland section of the orogen, i.e. in southeast Norway and southwest Sweden. The steep NW–SE strike of the HØDZ is also severely misoriented with regard to the dominating Devonian to Early Carboniferous NW–SE to E–W extension direction in western Norway (e.g. Fossen, Reference Fossen1992; Osmundsen & Andersen, Reference Osmundsen and Andersen2001).
In Late Devonian to Early Carboniferous times, the southern margin of Baltica was affected by the onset of the Variscan orogeny to the south (e.g. Kalani et al. Reference Kalani, Faleide and Gabrielsen2020; Fig. 8b). In the Saxo-Thuringian zone in the north of the orogen, initiation of convergence and collision is constrained between c. 380 and 360 Ma (Franke et al. Reference Franke, Cocks and Torsvik2017 and references therein). Along the southwestern margin of Baltica, this convergence was primarily expressed by significant dextral strike-slip deformation along NW–SE-striking faults and shear zones (Mazur et al. Reference Mazur, Aleksandrowski, Gągała, Krzywiec, Żaba, Gaidzik and Sikora2020), such as the Sorgenfrei–Tornquist zone and the HØDZ. We therefore speculate that the Late Devonian to Early Carboniferous 40Ar–39Ar plateau ages of the HØDZ pseudotachylites indicate one or several reactivation events caused by far-field stresses from the Variscan Orogeny (Fig. 8b). Variscan crustal shortening is also suggested as a possible tectonic source for the slickensided fracture planes at Forsmark (Tillberg et al. Reference Tillberg, Drake, Zack, Kooijman, Whitehouse and Åström2020), which was interpreted as sinistral strike-slip faults. The HØDZ, in contrast, may possibly have been active as a dextral transpressive fault zone due to its NW–SE orientation with regard to the assumed N–S-oriented Variscan shortening field (Fig. 8b). Although the kinematic framework of this deformation phase in the HØDZ needs to be better constrained, the interpretation may have consequences for the understanding of the larger Sorgenfrei–Tornquist zone. The initiation of this deformation zone is commonly attributed to Late Carboniferous dextral transtension (Erlström, Reference Erlström2020), but the new results presented herein suggest that it could have been formed in a transpressional setting already in the Late Devonian to Early Carboniferous (Fig. 8b).
6.c.5. Permian brittle reactivation events (Fig. 8c)
The tectonomagmatic evolution of the Oslo Rift is well constrained by relative and absolute dating of its igneous and early sedimentary components (Ramberg & Larsen, Reference Ramberg, Larsen, Dons and Larsen1978; Larsen et al. Reference Larsen, Olaussen, Sundvoll and Heeremans2008 a; Corfu & Larsen, Reference Corfu and Larsen2020). Age constraints on the structures controlling the localization of rifting are, however, lacking. The only exception is a K–Ar fault gouge pseudo-plateau age of c. 270–260 Ma from two N–S-trending faults in the Kongsberg Ag mines west of the Oslo Rift (Fig. 8c; Torgersen et al. Reference Torgersen, Viola, Zwingmann and Henderson2015 b). The c. 270 Ma pseudo-plateau age of the black fault gouge in the current study (BITE134; Figs 5, 7) documents that also the HØDZ, east of the rift, was reactivated at this time interval (Fig. 8c). Scarce kinematic data from these faults suggest dominating SE–NW to E–W extension, which is in accordance with the calculated Permo-Carboniferous palaeostress field of the Oslo Region (Sippel et al. Reference Sippel, Saintot, Heeremans and Scheck-Wenderoth2010). These faulting events correspond in time to the final stages of major magmatism and caldera collapses in the Oslo Rift (Larsen et al. Reference Larsen, Olaussen, Sundvoll and Heeremans2008 a; Corfu & Larsen, Reference Corfu and Larsen2020). Some small alkaline gabbros were intruded along N–S tectonic lineaments around 265 Ma (Neumann et al. Reference Neumann, Larsen and Sundvoll1985), which indicates that the faults also impacted on the localization of magmatism by acting as magma conduits.
The high-temperature-steps plateau age of SB009 pseudotachylite (294.6 ± 5.2 Ma; Fig. 6e) is a significant deformation age obtained in this study. It is interpreted to date the formation of pseudotachylites along the HØDZ, and its age is identical within error to the earliest recorded magmatism in the Oslo Rift (between 299 and 300 Ma; Corfu & Dahlgren, Reference Corfu and Dahlgren2008; Corfu & Larsen, Reference Corfu and Larsen2020). As such, it is the first geochronological documentation of deformation associated with the initial development of the Oslo Rift and shows that rifting was coupled with seismic activity (Fig. 8c). Curiously, the age is also identical within error to pseudotachylite 40Ar–39Ar laserprobe ages from the sinistral transtensional Møre–Trøndelag Fault Complex to the north of the Oslo Rift (Fig. 8c; Sherlock et al. Reference Sherlock, Watts, Holdsworth and Roberts2004).
Early–Mid Permian brittle deformation in Scandinavia is not limited to the Oslo Region. Similar faulting ages have also been documented in multiple K–Ar fault-dating studies in western Norway (Ksienzyk et al. Reference Ksienzyk, Wemmer, Jacobs, Fossen, Schomberg, Süssenberger, Lünsdorf and Bastesen2016; Viola et al. Reference Viola, Scheiber, Fredin, Zwingmann, Margreth and Knies2016; Fossen et al. Reference Fossen, Khani, Faleide, Ksienzyk and Dunlap2017; Scheiber & Viola, Reference Scheiber and Viola2018; Scheiber et al. Reference Scheiber, Viola, van der Lelij, Margreth and Schönenberger2019), and even in northern Scotland (Kemp et al. Reference Kemp, Gillespie, Leslie, Zwingmann and Campbell2019) and western Greenland (Rotevatn et al. Reference Rotevatn, Kristensen, Ksienzyk, Wemmer, Henstra, Midtkandal, Grundvåg and Andresen2018). It appears that this time interval represents an initial phase of widely distributed extension that is followed by the Late Permian to Early Triassic localized development of the northern North Sea rift and its counterpart in the Skagerrak graben (Fig. 8c; Gabrielsen et al. Reference Gabrielsen, Færseth, Steel and Kløvjan1990; Fossen et al. Reference Fossen, Ksienzyk, Rotevatn, Bauck and Wemmer2021). At a larger scale, the Early–Mid Permian marks a time interval where numerous rift systems developed throughout the newly assembled Pangaea supercontinent, eventually leading to the formation of the Neo-Tethys Ocean (e.g. Frizon de Lamotte et al. Reference Frizon de Lamotte, Fourdan, Leleu, Leparmentier and de Clarens2015).
6.c.6. Latest Triassic – Earliest Jurassic brittle reactivation (Fig. 8d)
The last datable activity along the HØDZ documented in our study is constrained by the overlapping ages of the finest size fraction in all three fault gouges at c. 200 Ma (Figs 7, 8d). This reactivation event must have been considerable since it involved authigenic mineral growth in all the HØDZ fault gouges. This argument is echoed on a larger scale by the fact that most of the dated fault gouges so far in southern Norway constrain fault activity around 200–150 Ma (Ksienzyk et al. Reference Ksienzyk, Wemmer, Jacobs, Fossen, Schomberg, Süssenberger, Lünsdorf and Bastesen2016; Viola et al. Reference Viola, Scheiber, Fredin, Zwingmann, Margreth and Knies2016; Scheiber et al. Reference Scheiber, Viola, van der Lelij, Margreth and Schönenberger2019; Tartaglia et al. Reference Tartaglia, Viola, van der Lelij, Scheiber, Ceccato and Schönenberger2020; Fossen et al. Reference Fossen, Ksienzyk, Rotevatn, Bauck and Wemmer2021; Hestnes et al. Reference Hestnes, Gasser, Scheiber, Jacobs, van der Lelij, Schönenberger and Ksienzyk2022), also including the Kongsberg Ag-mines faults at c. 200 Ma (Torgersen et al. Reference Torgersen, Viola, Zwingmann and Henderson2015 b). Similar to the Early–Mid Permian faulting phase, this phase of widespread brittle deformation also predates major rifting offshore (North Sea rift phase 2; Fig. 8d), leading Fossen et al. (Reference Fossen, Ksienzyk, Rotevatn, Bauck and Wemmer2021) to speculate whether this behaviour is a typical trait of continental rifting. It should be noted that the initiation of this Early Jurassic faulting phase around 200 Ma matches exactly with the emplacement of the Central Atlantic magmatic province (CAMP; Marzoli et al. Reference Marzoli, Callegaro, Dal Corso, Davies, Chiaradia, Youbi, Bertrand, Reisberg, Merle, Jourdan and Tanner2018 and references therein), which marks the start of the true disintegration of supercontinent Pangaea, according to Frizon de Lamotte et al. (Reference Frizon de Lamotte, Fourdan, Leleu, Leparmentier and de Clarens2015).
6.d. Fault localization and reactivation in plastic shear zones
The regional shear zones in the Sveconorwegian basement of southern Norway and Sweden are likely of lithospheric scale (e.g. Viola et al. Reference Viola, Henderson, Bingen and Hendriks2011; Bingen et al. Reference Bingen, Viola, Möller, Vander Auwera, Laurent and Yi2021), representing deformation zones that separate lithotectonic units with distinct geological histories. Important stages in the structural development of these deformation zones are preserved in the variety of partly overprinting deformation rocks that reveal shifting pressure- and temperature conditions and variation in stress field orientations. Once established, such structures tend to absorb repeated strain episodes independently of shifting P–T conditions and strain intensity (Watterson, Reference Watterson1975; Sibson, Reference Sibson1977; Nur, Reference Nur1982; Gabrielsen, Reference Gabrielsen1984; Gabrielsen & Braathen, Reference Gabrielsen and Braathen2014). The deformation geochronology presented herein shows that brittle deformation (i.e. Himdalen Fault) localized into and exploited the pre-existing Ørje Shear Zone throughout several distinct tectonic episodes (Fig. 8). This structural reactivation demonstrates that pre-existing lithospheric-scale zones of weakness may be preferentially reactivated whenever they are in a (semi-)favourable orientation in relation to the principal stress configuration.
Whereas the mechanisms that control repeated reactivation of brittle faults have been much studied (e.g. Sibson, Reference Sibson1985; Rutter et al. Reference Rutter, Holdsworth, Knipe, Holdsworth, Strachan, Magloughlin and Knipe2001; Collettini et al. Reference Collettini, Tesei, Scuderi, Carpenter and Viti2019), less is understood about the mechanisms that control the initiation of brittle deformation along pre-existing plastic shear zones. One hypothesis is that semi-continuous deformation across the brittle–plastic transition, such as in many large extensional detachment zones (e.g. Norton, Reference Norton1986; Fossen, Reference Fossen1992; Andersen, Reference Andersen1998; Braathen et al. Reference Braathen, Osmundsen and Gabrielsen2004), is required. However, the current study suggests that brittle deformation, represented by cataclasite, pseudotachylite and fault gouge, postdates the mylonitic fabric by more than 500 Ma (Fig. 8). Although we cannot exclude the possibility that minor cataclastic deformation was cogenetic with mylonitic shearing, it seems improbable that this should have had a major control on the brittle rheology of the shear zone.
Instead, we would highlight the fact that the brittle deformational rocks in HØDZ are almost perfectly parallel with the mylonitic fabric (Figs. 1–4), which leads us to suggest that the c. 908 Ma Ørje Shear Zone fabric was a lithospheric-scale mechanical anisotropy at the time of initial brittle deformation (c. 380–350 Ma; Fig. 8). The anisotropy was defined not only by quartz–felspathic segregation bands and parallel-oriented phyllosilicate planes (e.g. Fig. 3c–d), but also by alternating parallel-oriented phyllosilicate-rich lithologies such as phyllonites and sheared mafic dikes (Fig. 2). The importance of such mechanical anisotropies in controlling localization of brittle strain in plastic shear zones has been shown in field studies (e.g. Wehrens et al. Reference Wehrens, Baumberger, Berger and Herwegh2017), analogue experiments (e.g. Samsu et al. Reference Samsu, Cruden, Molnar and Weinberg2021), rock deformation experiments (e.g. Paterson & Wong, Reference Paterson and Wong2015) and numerical modelling (e.g. Vauchez et al. Reference Vauchez, Tommasi and Barruol1998; Naliboff et al. Reference Naliboff, Buiter, Péron-Pinvidic, Osmundsen and Tetreault2017). Wehrens et al. (Reference Wehrens, Baumberger, Berger and Herwegh2017) describe the phyllosilicate content and its spatial distribution as crucial for controlling the distribution of strain. This is in accordance with studies of phyllosilicate frictional properties, which are shown to be strongly dependent on their organization into throughgoing foliation planes (e.g. Collettini et al. Reference Collettini, Niemeijer, Viti and Marone2009; Tesei et al. Reference Tesei, Collettini, Carpenter, Viti and Marone2012; Haines et al. Reference Haines, Kaproth, Marone, Saffer and van der Pluijm2013). We therefore suggest that mechanical anisotropy of mylonitic fabrics is produced by parallel-oriented throughgoing phyllosilicate planes that can weaken the frictional strength of crustal-scale plastic shear zones on very long timescales. Crustal-scale shear zones may therefore impose a large-scale mechanical anisotropy on the strength of the crust, making them the most probable loci for accommodating subsequent brittle strain increments.
7. Conclusions
This structural–geochronological study of the Himdalen–Ørje Deformation Zone (HØDZ) uncovers the potentially long-lived, yet punctuated, structural evolution of geologically old, large-scale deformation zones. The study shows the importance of combining different geochronological methods with structural analysis and mineralogical constraints to characterize the evolution of such deformation zones. The main conclusions of this work are:
-
The HØDZ comprises a large range of deformation rocks from mylonite to fault gouge that reflects repeated structural reactivations during changing pressure-, temperature- and stress conditions.
-
The deformation zone was formed as a top-to-the-E compressional shear zone during the Mesoproterozoic Sveconorwegian orogeny, but the oldest geochronological data obtained in this study date late-Sveconorwegian, greenschist-facies top-to-the-W extensional reactivation at 908.6 ± 7.0 Ma (40Ar–39Ar white mica plateau age).
-
The first phase of brittle deformation is constrained between c. 380 Ma and c. 350 Ma by 40Ar–39Ar plateau ages of pseudotachylite veins and K–Ar ages of the coarsest size fractions in two fault gouges. We suggest that this event was caused by far-field stresses of the Variscan orogeny to the south.
-
Major structural reactivation of the HØDZ occurred during the development of the Permian Oslo Rift, as documented by K–Ar fault gouge dating (c. 270 Ma) and a 40Ar–39Ar pseudotachylite plateau age (294.6 ± 5.2 Ma). We interpret the latter to document seismic activity during the initiation of rift magmatism.
-
The last datable reactivation of the HØDZ was at c. 200 Ma, as recorded by K–Ar fault gouge dating. The age is similar to those derived from multiple other K–Ar fault-dating studies in southern Norway and testifies to a time interval of extensive, widespread overall E–W extension that predates major localized Jurassic–Cretaceous rifting offshore.
-
We have shown that it is possible to obtain geologically significant K–Ar ages from several generations of syn-kinematic authigenic illite within the same fault gouge if careful field sampling and structural characterization is combined with detailed mineralogical characterization, including illite crystallinity analysis.
-
We suggest that the main factor controlling the initiation of brittle deformation along pre-existing plastic shear zones is mechanical anisotropy defined by the presence of parallel-oriented, interconnected phyllosilicate foliation planes within the mylonitic fabric.
Supplementary material
To view supplementary material for this article, please visit https://doi.org/10.1017/S0016756822000966.
Acknowledgements
Jussi Mattila and Sam Haines are greatly thanked for their thorough and honest reviews that helped improve the quality of the article. We thank Kjetil Indrevær for initiating the collaboration, Alvar Braathen for commenting on the work and Anna Ksienzyk for sharing her national compilation of K–Ar fault gouge data. Ruikai Xie is thanked for careful K analysis.
Conflicts of interest
None.