1. Introduction
Malformed fossils present insight into how extinct forms responded to developmental, parasitic, and injury-related complications (Owen, Reference Owen1985; Babcock, Reference Babcock1993; Klompmaker et al. Reference Klompmaker, Kelley, Chattopadhyay, Clements, Huntley and Kowalewski2019; De Baets et al. Reference De Baets, Budil, Fatka, Geyer, De Baets and Huntley2022). Such specimens have been particularly useful for understanding facets of extinct arthropod groups (Babcock, Reference Babcock, Mikulic, Landing and Kluessendorf2003), including chelicerates, crustaceans, and trilobites (Owen, Reference Owen1985; Klompmaker et al. Reference Klompmaker, Karasawa, Portell, Fraaije and Ando2013; Bicknell et al. Reference Bicknell, Pates and Botton2018 c, Reference Bicknell, Pates, Kaiser, Zakrzewski, Botton, Tanacredi, Botton, Shin, Iwasaki, Cheung, Kwan and Mattei2022 b; Mitov et al. Reference Mitov, Dunlop and Bartel2021; De Baets et al. Reference De Baets, Budil, Fatka, Geyer, De Baets and Huntley2022). The most commonly documented malformed arthropod fossils are trilobites – a record that reflects their biomineralized exoskeleton (Babcock, Reference Babcock, Mikulic, Landing and Kluessendorf2003, Reference Babcock, Kelley, Kowalewski and Hansen2007; Fatka et al. Reference Fatka, Budil and Grigar2015; Bicknell et al. Reference Bicknell, Smith, Howells and Foster2022 d). This exoskeleton increases the preservational potential of individuals, and, by extension, improves the possibility of identifying malformed specimens.
Trilobites with malformations are often documented as stand-alone records, with examples ranging from the Cambrian through to the Carboniferous (Owen, Reference Owen1985; Babcock, Reference Babcock, Kelley, Kowalewski and Hansen2007; Bicknell et al. Reference Bicknell, Holmes, Pates, García-Bellido and Paterson2022 a). As such, few publications have presented a thorough record of malformations from the same deposit, especially considering the ratio of malformed individuals in the context of non-malformed specimens of the same species. However, over the last five years, there has been a transition to presenting detailed records of malformed specimens from the same deposit and contextualizing these specimens within a larger population (see Pates et al. Reference Pates, Bicknell, Daley and Zamora2017; Bicknell et al. Reference Bicknell, Paterson and Hopkins2019, Reference Bicknell, Holmes, Pates, García-Bellido and Paterson2022 a; Pates & Bicknell, Reference Pates and Bicknell2019; Bicknell & Smith, Reference Bicknell and Smith2022). To extend this line of enquiry, we considered Estaingia bilobata Pocock, Reference Pocock1964 from the Emu Bay Shale (Cambrian Series 2, Stage 4) of South Australia. This species is exceptionally abundant within the Emu Bay Shale Konservat-Lagerstätte, with the vast majority of specimens preserved as articulated exoskeletons (Paterson et al. Reference Paterson, García-Bellido, Jago, Gehling, Lee and Edgecombe2016; Holmes et al. Reference Holmes, Paterson and García-Bellido2021 a, b; Bicknell et al. Reference Bicknell, Holmes, Pates, García-Bellido and Paterson2022 a). The sheer abundance of specimens increases the likelihood of identifying malformed trilobites. Furthermore, injuries and pathologies are already known in other trilobites from the Emu Bay Shale and adjacent strata (Pocock, Reference Pocock1974; Conway Morris & Jenkins, Reference Conway Morris and Jenkins1985; Nedin, Reference Nedin1999; Paterson & Edgecombe, Reference Paterson and Edgecombe2006; Holmes et al. Reference Holmes, Paterson and García-Bellido2020; Bicknell et al. Reference Bicknell, Holmes, Pates, García-Bellido and Paterson2022 a). Here we document specimens of E. bilobata that exhibit injuries, pathologies, and teratologies, as well as evidence of possible durophagy (Figs 1–5). Furthermore, given the detailed information available on E. bilobata ontogeny (Holmes et al. Reference Holmes, Paterson and García-Bellido2021 a, b), we compare these specimens to a population of non-malformed individuals to illustrate at which size class malformed specimens occur, presenting unique insights into the palaeoecology of this abundant Emu Bay Shale trilobite.

Fig. 1. Estaingia bilobata with L-, U- and W-shaped injuries. (a, b) SAMA P46113. U-shaped injury to right side of thorax. (a) Complete specimen. (b) Close-up of injury (white arrow). (c, d) SAMA P45360. L-shaped injury to right side of thorax. (c) Complete specimen. (d) L-shaped injury showing recovering pleural spines (white arrows). (e, f) SAMA P52886. W-shaped injury to left side of thorax. (e) Complete specimen. (f) Close-up of injury (white arrow).
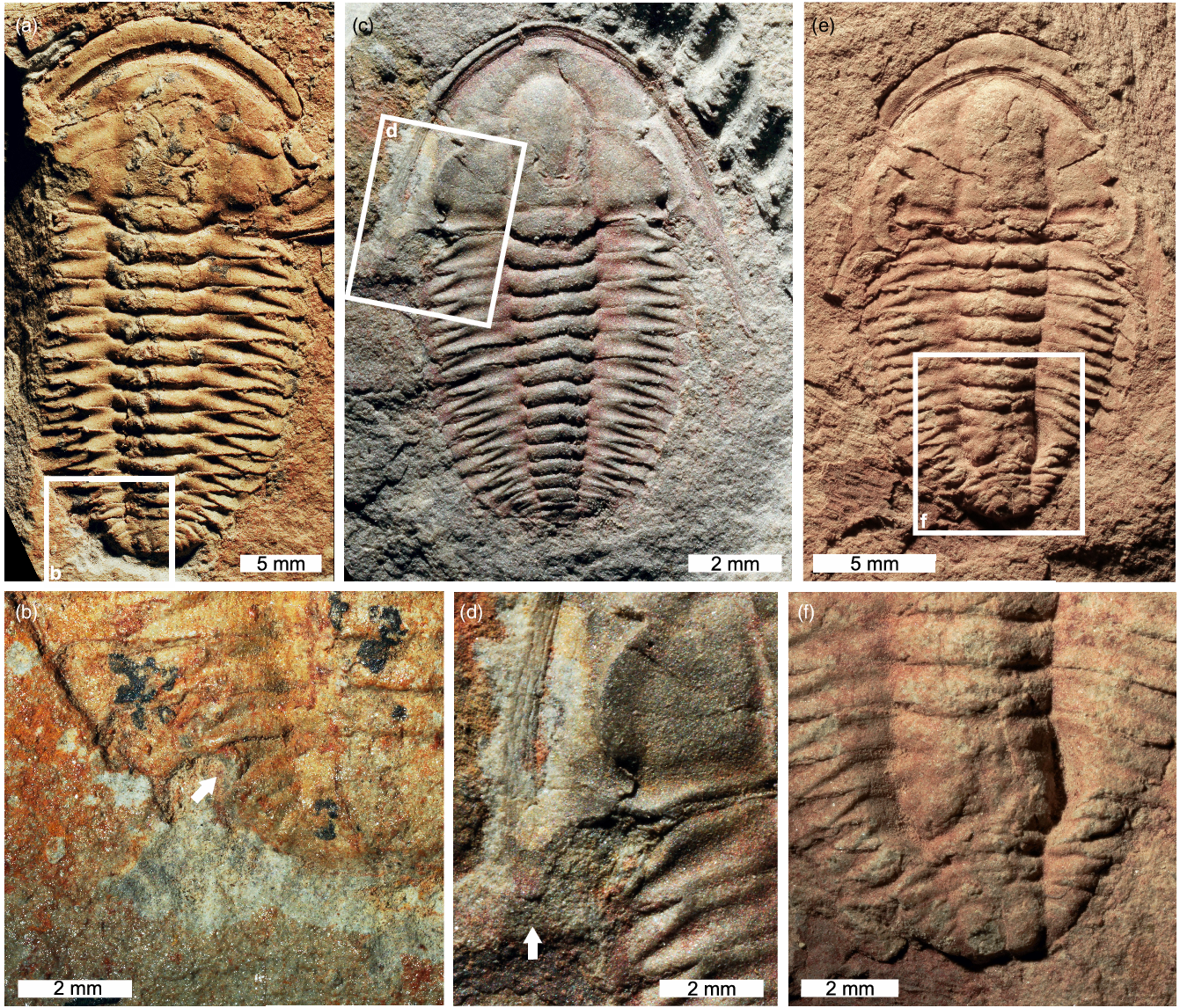
Fig. 2. Estaingia bilobata with cephalic and trunk injuries. (a, b) SAMA P46113. U-shaped injury to left side of pygidium. (a) Complete specimen. (b) Close-up of injury showing cicatrization (white arrow). (c, d) SAMA P52817. Stunted and rounded left genal spine. (c) Complete specimen. (d) Close-up of injury (white arrow). (e, f) SAMA P52892. Malformed and fused posterior thorax. (e) Complete specimen. (f) Close-up of injury.
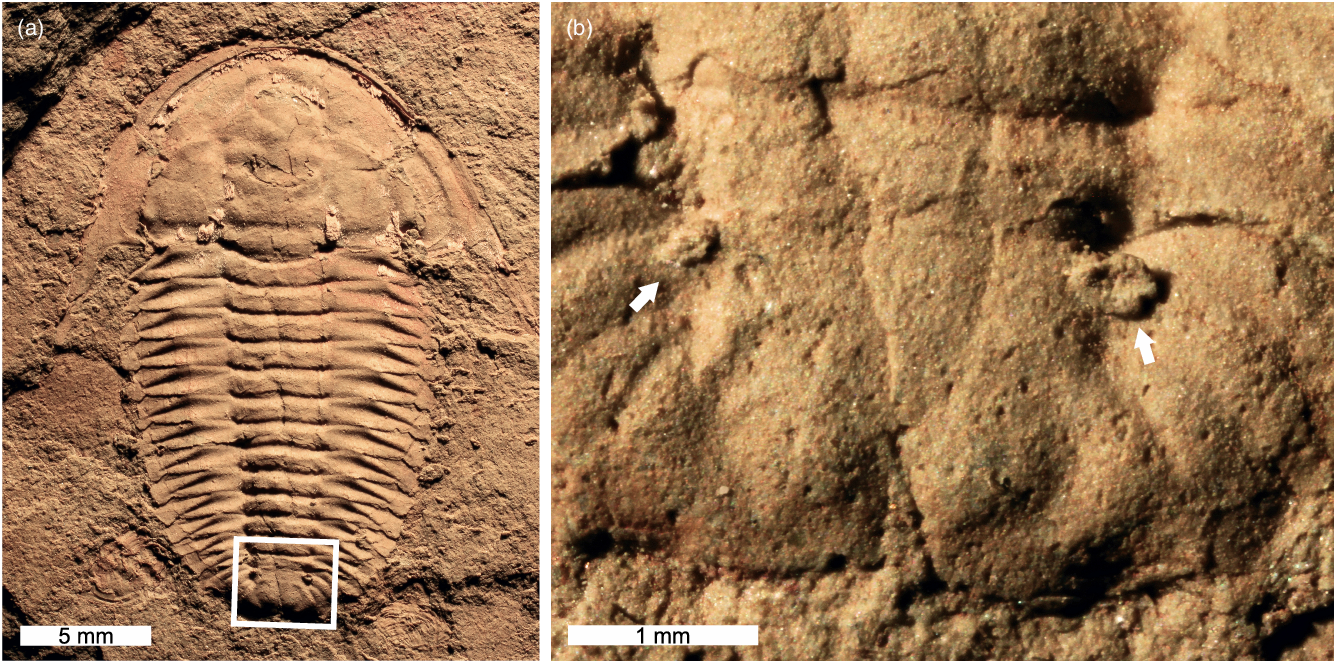
Fig. 3. Estaingia bilobata with two pathologies. (a, b) SAMA P59491. (a) Complete specimen. (b) Close-up of region with pathological neoplasms (white arrows).

Fig. 4. Estaingia bilobata with teratologies. (a, b) SAMA P54957. Fusion of axial rings (T5 and T6) and pleurae, some of which bifurcate distally. (a) Complete specimen. (b) Close-up of pleural bifurcations (white arrows). (c, d) SAMA P59487. Partial fusion of distal pleural sections. (c) Complete specimen. (d) Close-up of teratology (white arrows). (e, f) SAMA P59490. Additional ‘half-segment’. (e) Complete specimen showing asymmetrical trunk. (f) Close-up of teratology (white arrow).

Fig. 5. Examples of mangled Estaingia bilobata exoskeletons. (a, b) SAMA P46939. Shredded soft-shelled individual. (a) Part. (b) Counterpart. (c) SAMA P54820. Individual with severely broken and partially missing trunk. (d) SAMA P47975. Partial soft-shelled exoskeleton with torn thorax. (e) SAMA P54276. Soft-shelled individual with broken and partially missing trunk. (f) SAMA P 52867. Severely crushed exoskeleton with folded cephalon.
2. Geological and palaeoenvironmental context
The Emu Bay Shale forms part of the Kangaroo Island Group in the Stansbury Basin, which crops out on the north coast of Kangaroo Island (South Australia), and is interpreted as a nearshore delta complex (Gehling et al. Reference Gehling, Jago, Paterson, García-Bellido and Edgecombe2011; Jago et al. Reference Jago, Bentley, Paterson, Holmes, Lin and Sun2021). The Emu Bay Shale is considered to be c. 512 Ma (Pararaia janeae Zone; Cambrian Series 2, Stage 4) in age (Bengtson et al. Reference Bengtson, Conway Morris, Cooper, Jell and Runnegar1990; Paterson & Jago, Reference Paterson and Jago2006; Paterson & Brock, Reference Paterson and Brock2007; Jago et al. Reference Jago, Gehling, Betts, Brock, Dalgarno, García-Bellido, Haslett, Jacquet, Kruse and Langsford2020), based primarily on the co-occurrence of the emuellid trilobite Balcoracania dailyi Pocock, Reference Pocock1970 and an associated radiometric date of 511.87 ± 0.14 Ma in the Billy Creek Formation of the Arrowie Basin (Paterson & Edgecombe, Reference Paterson and Edgecombe2006; Paterson et al. Reference Paterson, Jago, Brock and Gehling2007; Betts et al. Reference Betts, Paterson, Jacquet, Andrew, Hall, Jago, Jagodzinski, Preiss, Crowley, Brougham, Mathewson, García-Bellido, Topper, Skovsted and Brock2018).
In the Big Gully area of Kangaroo Island, the Emu Bay Shale is 78 m thick at the shoreline and c. 61 m thick near Buck and Daily quarries, where the material considered here was sourced (c. 400 m inland; see García-Bellido et al. Reference García-Bellido, Paterson, Edgecombe, Jago, Gehling and Lee2009 for a detailed locality map). Here, the formation commences with a thin (up to 2 m) interval of polymict conglomerate, the base of which is interpreted as a sequence boundary separating it from the underlying Marsden Sandstone. Above this, the conglomerate transitions sharply into a package of dark grey, laminated mudstone with siltstone and fine sandstone event beds that become thicker and more common towards the contact with the sandstones of the overlying Boxing Bay Formation (Gehling et al. Reference Gehling, Jago, Paterson, García-Bellido and Edgecombe2011). The mudstone interval of the lower Emu Bay Shale is interpreted as representing a relatively deep-water prodelta environment, and hosts the Konservat-Lagerstätte that contains a diverse biota (50+ species, mainly within the basal c. 15 m of the formation), with many taxa considered to have been transported from shallower settings upslope, or having settled out of the water column (Paterson et al. Reference Paterson, García-Bellido, Jago, Gehling, Lee and Edgecombe2016). Exceptions include certain trilobite species, particularly the extraordinarily abundant Estaingia bilobata (with up to 600 individuals per square metre; JRP, unpublished data), as well as the redlichioids Redlichia takooensis Lu, Reference Lu1950 and R. rex Holmes, Paterson & García-Bellido, 2019 (Holmes et al. Reference Holmes, Paterson and García-Bellido2020). Intact moult configurations of these species suggest that they lived in or close to the low-oxygen environment of the prodelta setting, although were likely subject to occasional ‘mass kill’ events resulting from fluctuations of the oxycline (Paterson et al. Reference Paterson, García-Bellido, Jago, Gehling, Lee and Edgecombe2016). The prevalence of dorsum-down trilobite specimens in the deposit (up to 90% for E. bilobata; Drage et al. Reference Drage, Holmes, García-Bellido and Daley2018) may indicate a physiological response to oxygen stress.
3. Methods
All Estaingia bilobata specimens housed in the South Australian Museum (Adelaide, South Australia) palaeontological collection (SAMA P) were examined for evidence of malformations. Identified specimens were photographed under normal (fibre optic) lighting conditions using a Canon EOS 5D digital camera with a Canon MP-E 65 mm 1–5× macro lens.
The linear measurement dataset published by Holmes et al. (Reference Holmes, Paterson and García-Bellido2021 a) was used to determine where malformed Estaingia bilobata specimens were located in bivariate space compared to non-malformed specimens. The cephalic and trunk (thorax + pygidium) lengths in the dataset were used (Fig. 6a). Where possible, measurements from malformed specimens were added (Supplemental Information 1). Not all specimens documented here could be included due to partial preservation. Data were natural-log (ln) normalized and plotted. Data points were coded for malformation presence or absence.

Fig. 6. Measurements of Estaingia bilobata and bivariate plot showing distributions of malformations. (a) Reconstruction of E. bilobata showing measurements used in (b). (b) Bivariate space of E. bilobata. Most malformed specimens cluster with the largest specimens. Data found in Supplemental Data 1. Data were natural log normalized for plotting. Abbreviations: cl: cephalic length; tr: trunk (thorax + pygidium) length.
4. Terminology
4.a. Cicatrization
Exoskeletal thickening in a region where damage has occurred. This indicates an individual was alive and subsequently healed from the incurred injury (Rudkin, Reference Rudkin1979, Reference Rudkin1985; Babcock & Robison, Reference Babcock and Robison1989; Babcock, Reference Babcock1993, Reference Babcock, Mikulic, Landing and Kluessendorf2003).
4.b. Injury
Exoskeletal breakage through accidental injury, attack or moulting complications (sensu Bicknell et al. Reference Bicknell, Holmes, Pates, García-Bellido and Paterson2022 a). Injuries are usually L-, U-, V-, or W-shaped indentations across the exoskeleton (Babcock, Reference Babcock1993; Bicknell & Pates, Reference Bicknell and Pates2019; Bicknell et al. Reference Bicknell, Holmes, Pates, García-Bellido and Paterson2022 a). They can also be expressed as the reduction and rounding of exoskeletal sections, or as a ‘single segment injury’ (Bicknell et al. Reference Bicknell, Holmes, Pates, García-Bellido and Paterson2022 a, d). These features generally show cicatrization and/or segment repair and regeneration. Occasionally, injured exoskeletal areas recover abnormally, resulting in the fusion of exoskeletal sections and a lack of segment expression (Owen, Reference Owen1985; Bicknell et al. Reference Bicknell, Holmes, Pates, García-Bellido and Paterson2022 a). Injuries do not extend across the entire specimen – such breaks likely reflect post-mortem, taphonomic processes (Leighton, Reference Leighton, Laflamme, Schiffbauer and Dornbos2011).
4.c. Malformation
Evidence for injuries, teratologies, or pathologies (Owen, Reference Owen1985).
4.d. Pathology
Malformed exoskeletal sections resulting from parasitic activity or infections. These structures are often expressed as circular to ovate swellings (Šnajdr, Reference Šnajdr1978; Owen, Reference Owen1985; De Baets et al. Reference De Baets, Budil, Fatka, Geyer, De Baets and Huntley2022).
4.e. Teratology
External expressions of developmental, embryological, or genetic malfunctions (Owen, Reference Owen1985). These morphologies include addition or removal of nodes, segments, and spines, as well as abnormally developed morphologies (Owen, Reference Owen1985; Bicknell & Smith, Reference Bicknell and Smith2021).
5. Results
Examination of Estaingia bilobata specimens uncovered several examples of injuries, pathologies, and teratologies. We also found evidence for possible durophagous predation or post-mortem scavenging in the form of mangled exoskeletons.
5.a. Injuries
Six injured Estaingia bilobata are identified. Most injuries are unilateral, show L-, U-, W-shaped morphologies (Figs. 1, 2; Table 1) and are located on the trunk, showing evidence for pleural spine regeneration and rounding (Fig. 1c, d) and cicatrization (Figs 1e, f, 2a, b). However, there is a single specimen with a highly deformed posterior trunk showing fused thoracic segments (Fig. 2e, f). There is only one specimen that displays a cephalic injury, represented by a broken genal spine that has partially regenerated (Fig. 2c, d).
Table 1. Summary of injured Estaingia bilobata

5.b. Pathologies
Only one specimen shows evidence for pathological growths on the pygidium in the form of two ovate neoplasms (Fig. 3). Pathological growth on the right side of the pygidium is twice the size of the left neoplasm.
5.c. Teratologies
Three examples of teratologies are observed. The first shows abnormally developed posterior segments on the right side of the thorax, including the apparent fusion of certain axial rings (e.g. T5 and T6) and pleurae, some of which bifurcate distally (Fig. 4a, b). The second specimen displays very minor, partial fusion of distal sections of two anterior pleurae on the left side of the thorax (Fig. 4c, d). The third specimen has an additional ‘half-segment’ immediately behind T3 on the right side of the thorax. This additional segment begins at the sagittal line and rapidly expands to form the right half of an axial ring and a normal pleura (Fig. 4e, f). This has resulted in a highly asymmetrical trunk morphology, with the additional ‘half-segment’ also causing the posteriorly adjacent segment to bend.
5.d. Mangled exoskeletons
Five specimens with substantial exoskeletal breakage or ripping are noted (Fig. 5). The fully biomineralized specimens show extensive breakage over major exoskeletal regions (Fig. 5c, f). Specimens preserved in very low relief – interpreted as newly moulted, soft-shelled individuals (Drage & Daley, Reference Drage and Daley2016) – appear shredded or torn (Fig. 5a, b, e) and, in one case, shows complete removal of the cephalon and posterior trunk regions (Fig. 5d).
5.e. Bivariate space
The distribution of malformed Estaingia bilobata in bivariate space illustrates a clear overall size pattern (Fig. 6). The majority of malformed individuals cluster among the largest holaspid stages (Fig. 6b). The exceptions are two smaller specimens that are separate from other malformed individuals. There is no marked distinction between where injured, pathological, and teratological specimens are located in shape space.
6. Discussion
The specimens of Estaingia bilobata documented here show evidence for the three major groups of trilobite malformations (Owen, Reference Owen1985). This is rare among Cambrian trilobites, as the vast majority of malformed specimens record injuries, with limited evidence for teratologies and pathologies (Bergström & Levi-Setti, Reference Bergström and Levi-Setti1978; Bicknell & Paterson, Reference Bicknell and Paterson2018; De Baets et al. Reference De Baets, Budil, Fatka, Geyer, De Baets and Huntley2022). As such, E. bilobata presents a unique opportunity to study the palaeobiological significance of malformation types in one trilobite species from a single deposit.
The majority of observed Estaingia bilobata injuries (Figs. 1, 2a, b, e, f) are comparable to other documented examples of injured Cambrian trilobites (Rudkin, Reference Rudkin1979; Babcock, Reference Babcock1993; Bicknell & Pates, Reference Bicknell and Pates2020; Zong, Reference Zong2021 a, b). Indeed, select E. bilobata injuries are very similar to those observed on the co-occurring Redlichia takooensis and R. rex that have been attributed to failed predation (Conway Morris & Jenkins, Reference Conway Morris and Jenkins1985; Bicknell et al. Reference Bicknell, Holmes, Pates, García-Bellido and Paterson2022 a). It is highly likely that these injuries in E. bilobata also reflect failed predation. Evidence for cicatrization (Figs 1a, b, e, f, 2a, b) and regeneration (Fig. 1c, d) at injury sites indicates that these individuals survived and continued to moult after attacks. Other evidence for predation on, or post-mortem mastication of, E. bilobata is represented by the mangled exoskeletons (Fig. 5). Similar examples of R. takooensis have been interpreted as rare evidence of possible durophagy (e.g. Bicknell et al. Reference Bicknell, Holmes, Pates, García-Bellido and Paterson2022 a, fig. 1C–E). Such evidence of durophagy, combined with examples of failed predation, further bolsters the idea that E. bilobata was a key prey item within the Emu Bay Shale biota. This smaller trilobite species likely occupied a relatively low trophic level within the ecosystem (Jago et al. Reference Jago, García-Bellido and Gehling2016), especially given its extreme abundance (>80% of individuals across all known species), and yet injured specimens of E. bilobata are exceedingly rare. Further, most injured specimens cluster among the larger individuals in bivariate space. This may suggest that only larger individuals were capable of escaping and recovering from attacks, preserving records of failed predation. Alternatively, these specimens may preserve evidence of older injuries that recovered through subsequent moulting events. In either case, this pattern indicates a possible record of survivorship bias within the trilobite fossil record.
One injured specimen of Estaingia bilobata shows a substantially disrupted posterior thorax (Fig. 2e, f). This malformation is comparable to rare specimens of other Cambrian trilobite species with highly disrupted and wrinkled pleurae adjacent to the injury (Conway Morris & Jenkins, Reference Conway Morris and Jenkins1985; Bicknell et al. Reference Bicknell, Holmes, Pates, García-Bellido and Paterson2022 a). This condition is more commonly observed in the trunk of younger iso- and macropygous trilobites (Ormiston et al. Reference Ormiston, Logan and Fulton1967; Šnajdr, Reference Šnajdr1981; Owen, Reference Owen1985; Rudkin, Reference Rudkin1985). Disruption of pleurae indicates that the individual may have been attacked during the soft-shelled stage (shortly after moulting), resulting in a highly deformed exoskeleton that became fixed in subsequent moults (Conway Morris & Jenkins, Reference Conway Morris and Jenkins1985; Rudkin, Reference Rudkin1985).
Trilobite genal spine malformations are particularly rare (Owen, Reference Owen1985). Most malformed genal spines are represented by disrupted borders (e.g. Chatterton, Reference Chatterton1971; Cowie & McNamara, Reference Cowie and McNamara1978; Owen, Reference Owen1985; Babcock, Reference Babcock1993; Bicknell et al. Reference Bicknell, Pates and Botton2018 c), abnormal cephalic fringes (Owen, Reference Owen1983), or bifurcating genal spines (Owen, Reference Owen1985). Complete removal (Kay, Reference Kay1937) and recovery (Sinclair, Reference Sinclair1947; Hessin, Reference Hessin1988; Bicknell et al. Reference Bicknell, Smith, Bruthansová and Holland2022 c) of genal spines is exceedingly rare, as reflected by the single Estaingia bilobata specimen documented here. The documentation of only one genal spine injury (Fig. 2c, d) is noteworthy. Given the constrained anatomical region, a predator would unlikely have limited an attack to the genal area. As such, we can exclude failed predation as the most plausible explanation. Instead, the injury could reflect a complication during moulting or mechanical (non-predatory) damage that resulted in spine breakage (Owen, Reference Owen1983).
Teratological trilobite specimens with an additional ‘half-segment’ are almost completely unknown. To our knowledge, the only other example of an additional ‘half-segment’ within the thoracic region is a malformed Emuella polymera Pocock, Reference Pocock1970 that has multiple ‘half-segments’ (Pocock, Reference Pocock1974; Owen, Reference Owen1985; Paterson & Edgecombe, Reference Paterson and Edgecombe2006). One further record of this ‘half-segment’ condition is known from a Toxochasmops McNamara, Reference McNamara1979 pygidium from the Upper Ordovician (Katian) of Norway (Nielsen & Nielsen, Reference Nielsen and Nielsen2017). These rare teratologies likely record damage to a generative zone and the resultant propagation of additional, unaligned segments (Pocock, Reference Pocock1974; Owen, Reference Owen1985). It is noteworthy that two of the three examples represent early Cambrian forms, one of which (E. polymera) belongs to the family Emuellidae, which is characterized by unusually large numbers of trunk segments (Paterson & Edgecombe, Reference Paterson and Edgecombe2006). Emuellids in particular may have been more prone to teratologies as they added new segments through euanamorphosis (Paterson & Edgecombe, Reference Paterson and Edgecombe2006). These trilobites certainly had unusual patterns of trunk segment development compared with other forms (Holmes et al. Reference Holmes, Paterson and García-Bellido2021 a). This may have increased the likelihood of developmental malformations, indicating possible evidence for increased developmental plasticity within Cambrian trilobites compared to younger forms (Webster, Reference Webster2007). However, the rarity of these exceptional specimens suggests that these malfunctions seldom occurred.
Fused exoskeletal sections are rarely observed in thoracic pleurae (see Öpik, Reference Öpik1975, pl. 17), but more commonly recognized in pygidial regions (Strusz, Reference Strusz1980; Owen, Reference Owen1985; Babcock, Reference Babcock1993; Nielsen & Nielsen, Reference Nielsen and Nielsen2017; Bicknell & Smith, Reference Bicknell and Smith2021). Such teratologies have been attributed to genetic malfunctions (Nielsen & Nielsen, Reference Nielsen and Nielsen2017) and/or moulting complications producing a malformation that propagated in subsequent moults (Bicknell & Smith, Reference Bicknell and Smith2021). As the teratological thoracic segments (Fig. 4c, d) lack any evidence of an injury, this rare malformation was likely caused by a developmental abnormality that impacted a limited exoskeletal region. This may record segments failing to separate or articulate correctly when they budded off from the pygidium.
The morphologies of exceptionally preserved appendages in two Emu Bay Shale arthropod species have been linked to durophagy, potentially on Estaingia bilobata. Firstly, the short, stout gnathobasic spines on the biramous appendages of the very large trilobite Redlichia rex (Fig. 7e, f) have been shown to be comparable with other known durophages, such as the Cambrian Burgess Shale arthropod Sidneyia inexpectans Walcott, Reference Walcott1911 and the extant horseshoe crab Limulus polyphemus (Linnaeus, Reference Linnaeus1758) (Botton, Reference Botton1984; Bicknell et al. Reference Bicknell, Ledogar, Wroe, Gutzler, Watson and Paterson2018 a, b, Reference Bicknell, Holmes, Edgecombe, Losso, Ortega-Hernández, Wroe and Paterson2021, Reference Bicknell, Holmes, Pates, García-Bellido and Paterson2022 a; Holmes et al. Reference Holmes, Paterson and García-Bellido2020). This has permitted the linking of injuries identified on R. takooensis specimens with failed predation by the larger R. rex, and injuries to R. rex as possible instances of attempted cannibalism (Bicknell et al. Reference Bicknell, Holmes, Pates, García-Bellido and Paterson2022 a). Individuals of R. rex (and potentially R. takooensis, although morphological details of the appendages are unknown) may have also consumed E. bilobata, as supported by centimetre-scale coprolites containing E. bilobata remains (Daley et al. Reference Daley, Paterson, Edgecombe, García-Bellido and Jago2013, fig. 7E–H) that have been attributed to R. rex (Daley et al. Reference Daley, Paterson, Edgecombe, García-Bellido and Jago2013; Bicknell et al. Reference Bicknell, Holmes, Pates, García-Bellido and Paterson2022 a). The gnathobasic spines of the Emu Bay Shale chelicerate Wisangocaris barbarahardyae Jago et al., Reference Jago, García-Bellido and Gehling2016 (Fig. 7c, d) show similar morphologies to those of R. rex and other known durophages, and were likely also adapted for crushing biomineralized material (Jago et al. Reference Jago, García-Bellido and Gehling2016). This is supported by the presence of shelly cololites in certain specimens of W. barbarahardyae, some containing fragments of E. bilobata (Fig. 7a, b; Jago et al. Reference Jago, García-Bellido and Gehling2016, fig. 5c–g). Given the maximum reported size of W. barbarahardyae is c. 60 mm, this arthropod may have targeted and consumed E. bilobata meraspides. Juveniles of E. bilobata are abundant in the Lagerstätte interval and range from c. 1 to 5 mm in length (Holmes et al. Reference Holmes, Paterson and García-Bellido2021 a, b). This is consistent with the size of E. bilobata pleurae preserved within a W. barbarahardyae cololite (Fig. 7b). The size range and sheer abundance of E. bilobata within the Emu Bay Shale suggest that this small trilobite occupied an important position within the lower trophic levels of the local ecosystem, and was an important prey item for multiple species of predator.

Fig. 7. Examples of possible predators of Estaingia bilobata. (a–d) Wisangocaris barbarahardyae. (a, b) SAMA P55603a. Specimen showing shelly cololite containing E. bilobata fragments. (a) Near-complete specimen. (b) Close-up of cololite with sclerite fragments (white arrows). (c, d) SAMA P45629. Specimen showing series of gnathobases possibly used in durophagy of E. bilobata. (c) Near-complete specimen. (d) Close-up showing rows of gnathobasic spines. (e, f) SAMA P54942. Biramous appendage of Redlichia rex showing stout gnathobasic spines. (e) Complete specimen. (f) Close-up of stout gnathobasic spines.
Supplementary material
To view supplementary material for this article, please visit https://doi.org/10.1017/S0016756822001261
Acknowledgements
This research was funded by Australian Research Council grants (LP0774959, FT120100770 and DP200102005 to JRP and FT130101329 to DCGB), a National Geographic Society Research & Exploration grant (8991-11), a University of New England Postdoctoral Fellowship (to RDCB), a Karl Hirsch Memorial Grant (to RDCB), and a Royal Society of South Australia Research Grant (to RDCB). We thank Mary-Anne Binnie (SAMA) for access to, and help with collections. Finally, we thank Olev Vinn and an anonymous referee for their suggested changes to the manuscript.