1. Introduction
The Palaeoproterozoic Lomagundi–Jatuli Event was a major anomaly in the global cycling of carbon which records a positive excursion in δ13C composition in carbonates worldwide (Melezhik et al. Reference Melezhik, Huhma, Condon, Fallick and Whitehouse2007). This event was closely followed by the deposition of extensive black (carbon-rich) shales, the Shunga Event ˜2.0 Ga, on several continents (Condie et al. Reference Condie, Des Marais and Abbott2001; Strauss et al. Reference Strauss, Melezhik, Lepland, Fallick, Hanski, Filippov, Deines, Illing, Črne, Brasier, Melezhik, Prave, Hanski, Fallick, Lepland, Kump and Strauss2013; Martin et al. Reference Martin, Prave, Condon, Lepland, Fallick, Romashkin, Medvedev and Rychanchik2015). The abundance of carbon may reflect intense weathering of the continents following the Great Oxidation Event, and high productivity in the nutrient-rich oceans (Melezhik et al. Reference Melezhik, Fallick, Martin, Condon, Kump, Brasier and Salminen2013). The identification of this episode as the peak of black shale sedimentation in the Precambrian, from 2.0 to 1.85 Ga (Condie et al. Reference Condie, Des Marais and Abbott2001), is based on data from Australia (57 %), North America (37 %) and Russia (6 %). New exploration for graphite deposits, required for industrial processes, batteries and possibly manufacture of graphene, has drawn attention to many additional successions of Palaeoproterozoic black shale, now metamorphosed to graphitic metasediments, and the unique nature of this carbon burial episode.
The record of graphite as a measure of Palaeoproterozoic carbon burial is expressed here as (i) a review of the depositional ages of the biggest graphite deposits, (ii) a compilation of carbon/sulphur elemental datasets for graphitic metasediments and (iii) a review of carbon isotope compositions of Palaeoproterozoic graphite. Combined, the data will confirm if the Palaeoproterozoic was a period of anomalous carbon burial, if the carbon was derived from sedimentary organic matter rather than carbonic fluids during metamorphism, and if the carbon caused burial of sulphur as in younger sediments.
2. Methods
Stable carbon isotope analysis was conducted at SUERC on 20 graphitic samples digested in 10 % HCl overnight to remove trace carbonate. Samples were analysed by standard closed-tube combustion method by reaction in vacuo with 2 g of wire form CuO at 800 °C overnight. Data are reported in per mil (‰) using the δ notation versus Vienna Pee Dee Belemnite (V-PDB). Repeat analysis of laboratory standard gave δ13C reproducibility around ±0.2 ‰ (1 s). Other isotopic data were collated from cited literature. Total organic carbon (TOC) and S contents were measured using a LECO CS225 elemental analyser at the University of Aberdeen, with standards 501-024 (Leco Instruments, instrument uncertainty ±0.05 % C, ±0.002 % S) and BCS-CRM 362. Data are reported on a carbon/sulphur cross-plot, relative to the modern marine composition (Berner & Raiswell, Reference Berner and Raiswell1983). The datasets reported are for rock units with TOC levels above 1 wt %, i.e. they are classified as black shales (TOC >0.5 wt %; Huyck, Reference Huyck1990). The repeatability, based on three repeats of CRMs and blanks, was consistently within 1 wt %. Collation of the world’s largest graphite deposits was performed using a typical industry cut-off for exploitation of 8 wt % carbon (data file available from the authors). Tonnages of ore at major ore deposits were collated from cited literature. The long-term legacy of the Palaeoproterozoic graphitic rocks for mineralization that is important to global metal resources is demonstrated by reviews herein of nickel (+cobalt–copper–PGE (platinum-group element)) mineralization, which occurs in mafic/ultramafic intrusions; and unconformity-related uranium mineralization.
3. Results
3.1. Anomalous Palaeoproterozoic carbon reservoir
Of the 33 richest graphite deposits in the world (minimum 8 wt % carbon), 28 of 33 (85 %) deposits are of Palaeoproterozoic age (Fig. 1; Table S1, in the Supplementary Material available online at https://doi.org/10.1017/S0016756821000583). Most of the deposits are dated to the range 2.1–1.85 Ga. Graphite deposits not dated to this interval are either Palaeoproterozoic but not so precisely dated, or Archaean. Hydrocarbon source rocks in younger successions are attributed excellent organic richness at mean contents above 4 wt % carbon, confirming the exceptional nature of the Palaeoproterozoic carbon accumulation. Younger episodes of high carbon accumulation, including in the late Neoproterozoic (Condie et al. Reference Condie, Des Marais and Abbott2001), were less extensive and thus had less opportunity to influence subsequent processes such as mineralization. The rich graphite deposits are predominantly vein-type, but there are many non-vein deposits of lower grade, and also younger less rich vein deposits (Robinson et al. Reference Robinson, Hammarstrom and Olson2017).

Fig. 1. World’s richest graphite ore deposits. Deposits ranked by mean carbon content (wt %), collated from publicly available technical reports for exploration projects (Table S1, in the Supplementary Material available online at https://doi.org/10.1017/S0016756821000583). Data shown adopt a lower cut-off of 8 % carbon, commonly used in graphite exploration. Where clusters of several deposits occur together (e.g. at Balama), largest deposit is plotted. Palaeoproterozoic deposits highlighted and account for 28 of 33 richest deposits. Map shows global distribution of the richest graphite ores.
3.2. Sulphur contents of graphitic sediments
The cross-plot of organic carbon and sulphur (Fig. 2; Table S2, in the Supplementary Material available online at https://doi.org/10.1017/S0016756821000583) shows that in multiple datasets the S/C ratio is greater than the modern marine sediment value of c. 0.35. This is similarly reported in Palaeoproterozoic shales by Paiste et al. (Reference Paiste, Pellerin, Zerkle, Kirsimäe, Prave, Romashkin and Lepland2020). The direct implication is that the anomalously high carbon burial had the consequence of causing anomalously high sulphur sequestration in many Palaeoproterozoic successions. The carbon contents may be lower than originally deposited due to volatile loss during metamorphism (Raiswell & Berner, Reference Raiswell and Berner1987), but correction for this would imply even richer carbonaceous deposits at the time of deposition.
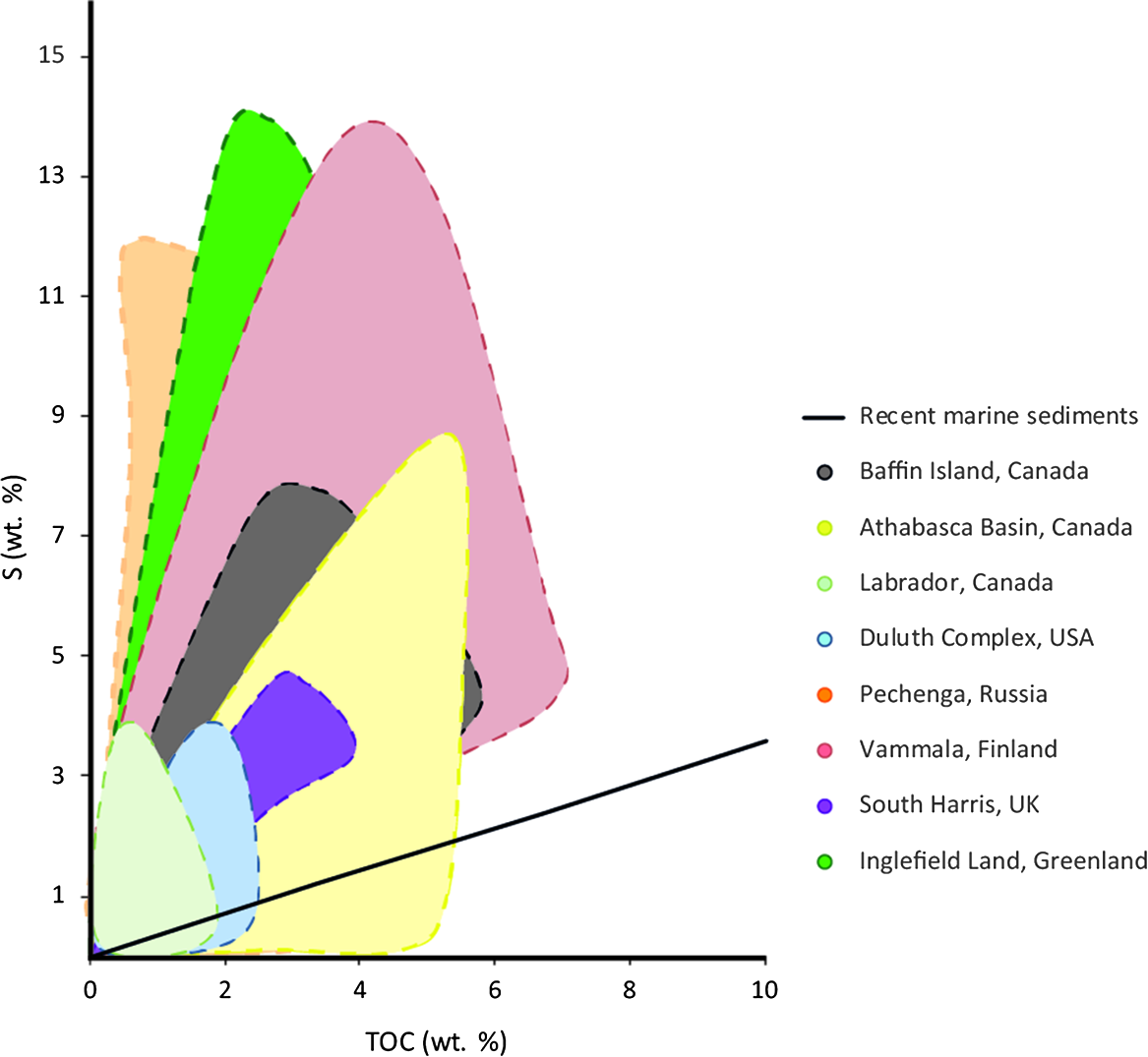
Fig. 2. Cross-plot of sulphur and organic carbon contents for Palaeoproterozoic country rocks for younger ore deposits and prospects. Reference line for recent marine sediments from Berner & Raiswell (Reference Berner and Raiswell1983). S/C ratios for these deposits are consistently higher than the line, reflecting extensive pyrite formation that would enhance mineralization during subsequent intrusion. Datasets (Table S2, in the Supplementary Material available online at https://doi.org/10.1017/S0016756821000583) from Andrews & Ripley (Reference Andrews and Ripley1989), Peltonen (Reference Peltonen1995), Melezhik et al. (Reference Melezhik, Grinenko and Fallick1998), Ripley et al. (Reference Ripley, Li and Shin2002), Partin et al. (Reference Partin, Bekker, Planavsky and Lyons2015), Pascal et al. (Reference Pascal, Ansdell, Annesley, Potter and Wright2015) and this study (Greenland, UK).
Some Palaeoproterozoic black shales do not show a concomitant sulphur enrichment (e.g. de Putter et al. Reference De Putter, Liégeois, Dewaele, Cailteux, Boyce and Mees2018), but most are sulphur-rich. While the shales are typically sulphur-rich, beds of mineable graphite are not sulphur-rich, and would not be commercially viable if they were (Lu & Forssberg, Reference Lu and Forssberg2002; Chelgani et al. Reference Chelgani, Rudolph, Kratzsch, Sandmann and Gutzmer2016).
3.3. Isotopic composition of Palaeoproterozoic graphite
As many of the graphite deposits were metamorphosed to amphibolite or granulite facies, it is feasible that the original sedimentary protolith could be supplemented by graphite precipitated from volatile solutions containing CO2, H2O and CH4 during metamorphism, which should be evident in heavier carbon isotope values (Huizenga & Touret, Reference Huizenga and Touret2012; Luque et al. Reference Luque, Crespo-Feo, Barrenechea and Ortega2012). Data for 31 graphite mines and prospective deposits in rocks of Palaeoproterozoic age (Fig. 3; Table S3, in the Supplementary Material available online at https://doi.org/10.1017/S0016756821000583), from 15 countries distributed globally, are consistently in the range −15 to −37 ‰. This range indicates an origin exclusively from organic matter, and not enriched from a carbonate source during metamorphism. Two separate databases for Palaeoproterozoic kerogen (Krissansen-Totton et al. Reference Krissansen-Totton, Buick and Catling2015; Havig et al. Reference Havig, Hamilton, Bachan and Kump2017) confirm that organic matter of this age had δ13C −35 to −20 ‰. The graphite therefore represents the anomalous Palaeoproterozoic deposition of organic carbon. By contrast, several graphite deposits deposited from volatile CO2-rich solutions in Precambrian granulites have a range of −18 to −5 ‰ (Vry et al. Reference Vry, Brown, Valley and Morrison1988; Huizenga & Touret, Reference Huizenga and Touret2012; Luque et al. Reference Luque, Crespo-Feo, Barrenechea and Ortega2012; Buseck & Beyssac, Reference Buseck and Beyssac2014). There are examples of graphite in Palaeoproterozoic marbles (limestones) with relatively heavy compositions (e.g. Garde, Reference Garde1979), in which the graphite must be at least partially derived from decarbonation. However, these are minor showings, which do not impact on our dataset of rich graphite deposits.

Fig. 3. Carbon isotopic compositions of Palaeoproterozoic graphite mines and prospective deposits, and three Ni ore deposits in Palaeoproterozoic rocks. Data from 15 countries, listed in Table S3, in the Supplementary Material available online at https://doi.org/10.1017/S0016756821000583. Data from graphite are similar to data from organic matter (kerogen) for period 2.0–1.5 Ga (Krissansen-Totton et al. Reference Krissansen-Totton, Buick and Catling2015; Havig et al. Reference Havig, Hamilton, Bachan and Kump2017). Precambrian graphite precipitated from carbon dioxide has distinct range of compositions (Luque et al. Reference Luque, Crespo-Feo, Barrenechea and Ortega2012).
3.4. Mineralization
Large nickel (+Co–Cu–PGE) deposits occur in mafic–ultramafic cumulate bodies, many of which were intruded through Palaeoproterozoic graphitic rocks (Hoatson et al. Reference Hoatson, Jaireth and Jaques2006). Assimilation of sulphur- and/or carbon-rich strata was critical to mineralization, as it caused sulphur saturation and sulphide precipitation in the magma. Assimilation is supported by sulphur isotope data (Ripley, Reference Ripley2014). The host rocks to Ni (+Co+Cu+PGE) intrusions have high S/C ratios typical of anoxic/euxinic sediments (Berner & Raiswell, Reference Berner and Raiswell1983), and the high carbon contents are thus associated locally with abundant pyrite and pyrrhotite (Fig. 2). The sulphide-bearing graphitic hosts also contributed trace elements to the melt that promoted mineralization. For example, tellurium incorporated into pyrite in anoxic sediments became concentrated by assimilation into melts where it could precipitate PGE and gold tellurides (Peltonen, Reference Peltonen1995; Samalens et al. Reference Samalens, Barnes and Sawyer2017). Almost all of the largest post-Archaean nickel deposits are either of Palaeoproterozoic age, or they are younger but were intruded through and assimilated Palaeoproterozoic strata (Fig. 4). The assimilation of carbonaceous (and sulphidic) material is evidenced by xenoliths of metasediment, and by neoformed graphite crystallized from contaminated melt. Thus, graphite occurs in the super-large deposits formed at Voisey’s Bay at 1.33 Ga, and Duluth at 1.1 Ga, and graphitic marble xenoliths occur in Jinchuan at 0.85 Ga, all following emplacement through graphitic Palaeoproterozoic rocks. At Duluth, the dependence of mineralization on assimilated carbon is especially evident from the distribution of ore bodies at the margin between the Duluth Complex intrusion and the host carbonaceous shales (Ripley et al. Reference Ripley, Li and Shin2002; Ripley, Reference Ripley2014). Nickel and PGEs in these intrusions are commonly accompanied by cobalt, and some of the highest-tonnage bedrock cobalt resources are in intrusions which had assimilated Palaeoproterozoic graphitic sediment, including the younger intrusions at Voisey’s Bay and Duluth (Slack et al. Reference Slack, Kimball and Shedd2017) (Fig. 4). Unconformity-hosted deposits are the biggest producers of uranium ores. The highest-yielding deposits, in Canada and Australia, occur at unconformities between a Mesoproterozoic cover succession and a Palaeoproterozoic substrate. The substrate is consistently graphitic, especially in shear zones, and the graphite is attributed a role as a reductant and locus of high heat flow (Jefferson et al. Reference Jefferson, Thomas, Gandhi, Ramaekers, Delaney, Brisbin, Cutts, Quirt, Portella, Olson and Goodfellow2007) or source of reducing methane (Branquet et al. Reference Branquet, Boulvais, Mercadier, Ledru and Khairallah2019), although there is debate about whether the graphite is essential or not. While the Palaeoproterozoic graphite is 1.8 Ga or older, the uranium mineralization which it influenced may be substantially younger. Deposits in Canada and Australia were first mineralized at 1.59 Ga and 1.68 Ga respectively, but were reactivated at intervals up to the Phanerozoic (Jefferson et al. Reference Jefferson, Thomas, Gandhi, Ramaekers, Delaney, Brisbin, Cutts, Quirt, Portella, Olson and Goodfellow2007). Elsewhere, graphite-bearing strata of Palaeoproterozoic age were mineralized over a billion-year period at 1.4 Ga in European Russia (IAEA, 2018), 1.23 Ga in the Russian Pacific (Goroshko & Gur’yanov, Reference Goroshko and Gur’yanov2007), ˜1.2 Ga in Rio Preto, Brazil (De Figueiredo Filho, Reference De Figueiredo Filho1984), 1.12 Ga in Shillong, India (Awati et al. Reference Awati, Harikrishnan, Sinha, Gupta and Gupta1995), and 0.8 Ga in Kintyre, Western Australia (IAEA, 2018). Of the 23 largest unconformity-related uranium ore systems, the largest 13, and 20 of the 23, are associated with Palaeoproterozoic graphite (Fig. 4), accounting for 97.5 % of the total tonnage (IAEA, 2018). Despite models in which the graphite is not essential to mineralization (Jefferson et al. Reference Jefferson, Thomas, Gandhi, Ramaekers, Delaney, Brisbin, Cutts, Quirt, Portella, Olson and Goodfellow2007), this represents a very high proportion. In addition to uranium, some of these unconformity-related deposits also yield platinum group elements, gold, selenium and tellurium (Davidson & Large, Reference Davidson and Large1994).

Fig. 4. High metal tonnages from mineralized intrusions and unconformities associated with Palaeoproterozoic graphitic hosts. (a) Nickel tonnages for largest deposits, modified from Hoatson et al. (Reference Hoatson, Jaireth and Jaques2006), excluding impact-related Sudbury deposit. All non-Archaean deposits were intruded into carbonaceous rocks, and almost all into Palaeoproterozoic carbonaceous hosts. (b) Cobalt tonnages for largest deposit types in bedrock, omitting laterite and seafloor (Slack et al. Reference Slack, Kimball and Shedd2017). Individual intrusions through Palaeoproterozoic carbonaceous hosts identified. (c) Unconformity-related uranium ore systems, expressed as cumulative mass of the 23 largest systems (IAEA, 2018), showing 97.5 % are associated with Palaeoproterozoic graphite.
In summary, the ages of the ore deposits show that both the cumulate-hosted nickel and unconformity-related uranium mineralization occur 200 and more million years after deposition of the graphite (Fig. 5), i.e. the Palaeoproterozoic graphite has a long-term metallogenic legacy.

Fig. 5. Ore deposits genetically linked to Palaeoproterozoic graphite but formed at least 200 million years since graphite deposition. (a) Examples through time of ore deposits, in which the graphite influenced ore deposition for 2 billion years. (b) Global distribution of Proterozoic rocks, and examples of younger ore deposits associated with Palaeoproterozoic graphite beds.
4. Discussion
4.1. High carbon contents
The very high organic richness of Palaeoproterozoic black shales (Condie et al. Reference Condie, Des Marais and Abbott2001) is matched by the very high mean carbon contents and abundance of commercial graphite deposits of Palaeoproterozoic age. Critically, the high carbon accumulation during the Palaeoproterozoic coincided with a relatively high crustal preservation potential, related to supercontinent assembly (Condie et al. Reference Condie, Des Marais and Abbott2001; Condie, Reference Condie2014), leading to the long-term survival and mineralizing legacy of the Palaeoproterozoic carbon reservoir. The black shale data are derived from a geographically limited region (Australia, North America, Russia), but the graphite data represent several additional continents. Recent data include much information from Scandinavia, Ukraine, Brazil, India, China and several parts of Africa, and so confer much greater reliability as a global signature, and thus also support the global nature of the coupled Lomagundi–Jatuli and Shunga events.
The carbon isotope data for Palaeoproterozoic graphite, indicating an origin in sedimentary organic matter, link the graphite to the host sequences. In many cases, the graphitic beds have a bedding-parallel form that suggests that they represent layers in the original succession, for example in Palaeoproterozoic successions in South Australia (Keeling, Reference Keeling2017) and North Norway (Gautneb et al. Reference Gautneb, Rønning, Engvik, Henderson, Larsen, Solberg, Ofstad, Gellein, Elvebakk and Davidsen2020). The graphite commonly occurs in close proximity to non-commercial, but nonetheless highly graphitic, beds that are unequivocally metasediments. However, several lines of evidence indicate that the graphite beds were modified from the deposited beds. The graphite is commonly replacive, i.e. graphite overprinted other minerals, and graphite shows structurally-controlled thickening such as in fold hinges (e.g. Gautneb et al. Reference Gautneb, Rønning, Engvik, Henderson, Larsen, Solberg, Ofstad, Gellein, Elvebakk and Davidsen2020). Furthermore, ore graphite can be less crystallographically ordered than graphite in the background succession, as measured by Raman spectroscopy (e.g. Kríbek et al. Reference Kríbek, Sýkorová, Machoviⓒ, Knésl, Laufek and Zachariáš2015), showing that it was mobilized and/or modified. The distinction between high-sulphur graphitic metasediments and low-sulphur graphite deposits indicates a marked change in composition. Even in successions which contain sulphide ore mineralization in graphitic rocks, such as at Talvivaara, Finland (Kontinen & Hanski, Reference Kontinen, Hanski, Maier, Lahtinen and O’Brien2015), and the Bhilwara/Aravalli supergroups, India (Mishra & Bernhardt, Reference Mishra and Bernhardt2009), the commercial graphite prospects have low sulphur contents. This implies that carbon and sulphur had become fractionated during metamorphism, and is consistent with models in which graphitic sediments release large volumes of sulphur-rich fluids during metamorphism (Poulson & Ohmoto, Reference Poulson and Ohmoto1989; Tomkins, Reference Tomkins2010).
4.2. Mineralization in Palaeoproterozoic rocks
Given the reductive properties of organic carbon, and the fundamental role of redox processes in metallogeny, a consequence of this episode of anomalous carbon burial was a step change in metal sequestration both during the Palaeoproterozoic and during subsequent geological history. The importance of the Palaeoproterozoic deposition of carbon to global metallogeny is evident by considering the diversity of metal ores precipitated, the tonnages of the ores, and the continued influence of the carbon through geologic time. Palaeoproterozoic rocks were extensively mineralized at the time of deposition, including iron formations and manganese mineralization both deposited from anoxic seawater and interbedded with graphitic rocks: about 65 % of global manganese reserves were deposited in the Palaeoproterozoic over 2.3–1.8 Ga (Havig et al. Reference Havig, Hamilton, Bachan and Kump2017; De Putter et al. Reference De Putter, Liégeois, Dewaele, Cailteux, Boyce and Mees2018). However, a legacy of continued long-term mineralization was conferred by the physico-chemical nature of the graphitic beds. Firstly, the highly ductile nature of the graphite made it a focus for deformation and in particular the formation of shear zones, which channelled younger mineralizing fluids. Secondly, the conductive nature of the graphite may have engendered heat flow anomalies that caused fluid circulation. Thirdly, the reductive power of concentrated organic carbon made the graphite a chemical trap to pull metals out of solution. Fourthly, the carbon could be consumed in chemical reactions that precipitated metals. Fifthly, graphitic sediments are commonly sulphidic, as expected in marine shales, and sulphides co-precipitate trace elements during early diagenesis. These properties allowed a wide range of ores, involving different metals, to precipitate in Palaeoproterozoic graphitic rocks (Pirajno et al. Reference Pirajno, Thomassen and Dawes2003; Jefferson et al. Reference Jefferson, Thomas, Gandhi, Ramaekers, Delaney, Brisbin, Cutts, Quirt, Portella, Olson and Goodfellow2007; Williams, Reference Williams2007).
The distributions of the deposit types through time have different controlling factors. The uranium in unconformity-hosted deposits was derived particularly from the erosion of granites aged from Archaean to Mesoproterozoic. This uranium became available before younger episodes of carbon precipitation and so is preferentially associated with crust rich in Palaeoproterozoic carbon. In the case of Ni–Co–Cu–PGE, even where the host intrusion is much younger than Palaeoproterozoic, where the intrusion cuts through the Palaeoproterozoic it may host ores formed by assimilation of Palaeoproterozoic graphite and sulphide. The high preservation potential of Palaeoproterozoic strata (Condie et al. Reference Condie, Des Marais and Abbott2001; Condie, Reference Condie2014) has contributed to a dominance of country rocks of this age.
4.3. Mineral exploration
Much mineralization occurs in Archaean and Palaeoproterozoic rocks (Groves et al. Reference Groves, Vielreicher, Goldfarb, Condie, McDonald, Boyce, Butler, Herrington and Polya2005). This was followed by the so-called ‘boring billion’ period (1.8 to 0.8 Ga) when a combination of lower crustal growth and low preservation potential limit the mineralization record. Consequently, ore exploration occurs especially in Canada, Brazil, Australia, Scandinavia and North China, where much bedrock is Archaean and (Palaeo)proterozoic. Mineral exploration in these rocks has emphasized the importance of Palaeoproterozoic graphite. Fundamentally, graphitic rocks have guided the search for unconformity-related uranium in many countries (IAEA, 2018) and thus graphite has become an exploration target.
Exploration for several types of ore deposit makes use of the high electrical conductivity of the graphite. This allows the search for, and delineation of, individual ore deposits in the subsurface, for example deposits of unconformity-related uranium (Tuncer et al. Reference Tuncer, Unsworth, Siripunvaraporn and Craven2006). However, graphite in Palaeoproterozoic successions is so abundant that it can be detected by geophysical techniques at the basin scale (Boerner et al. Reference Boerner, Kurtz and Craven1996; Zhamaletdinov, Reference Zhamaletdinov1996; Lindsay et al. Reference Lindsay, Spratt, Occhipinti, Aitken, Dentith, Hollis, Tyler, Gessner, Blenkinsop and Sorjonen-Ward2018). This abundance is not evident in rocks of younger ages, and the data evidences a substantial critical reservoir of graphite of Palaeoproterozoic age.
The strong link between metal ores and Palaeoproterozoic graphite is evident in frontier exploration areas where individual mining companies explore for both graphite and metals in adjoining or overlapping licences, including in Canada, Scandinavia, Africa and Australia (Table S4, in the Supplementary Material available online at https://doi.org/10.1017/S0016756821000583). Both ore types are essential to future, environmentally sensitive technologies (Gautneb et al. Reference Gautneb, Knežević, Gloaguen, Melleton, Gourcerol and Törmänen2019). Most commonly, companies target both graphite and nickel ores, but graphite is also sought along with copper, uranium, gold and iron. This recent trend in exploration is likely to lead to discoveries which further demonstrate the strong link between Palaeoproterozoic graphite and metalliferous mineralization.
5. Conclusions: unique importance of Palaeoproterozoic carbon
The abundance of the world’s largest graphite deposits that are of Palaeoproterozoic age supports previous inferences that the Palaeoproterozoic was a period of anomalously high carbon burial. The high S/C ratios for many Palaeoproterozoic shales show that, in addition to carbon, this was a period of high sulphide burial. The combined carbon and sulphur conveyed a reductive character to the sediments which gave them exceptional mineralizing potential.
Criteria for assessing the importance of Palaeoproterozoic carbon to mineralization, i.e. diversity of ores, tonnages of ores, and longevity of influence, all indicate a unique role for Palaeoproterozoic carbon in Earth’s geological history. While there were episodes of deposition of single metals with greater tonnage, such as Archaean banded iron formations, longevity in the Palaeoproterozoic rocks was unequalled. The Palaeoproterozoic deposition of carbonaceous sediment had a greater control on the long-term metallogeny of the planet than any other depositional episode in the planet’s history.
Declaration of Interest
The authors declare none.
Acknowledgements
This project is in support of the Natural Environment Research Council SoS (Security of Supply of Critical Elements) programme, under Grant NE/M010953/1. C Taylor, J Johnston and J Bowie provided skilled technical help. We are most grateful to H Gautneb and E Lynch for valuable review.
Supplementary material
To view supplementary material for this article, please visit https://doi.org/10.1017/S0016756821000583.