1. Introduction
The Toarcian Oceanic Anoxic Event (T-OAE) is generally regarded as a global, short-lived perturbation of the carbon cycle that occurred during the Exaratum Subzone of the Falciferum Zone (Jenkyns, Reference Jenkyns1988; Hesselbo et al. Reference Hesselbo, Grocke, Jenkyns, Bjerrum, Farrimond, Morgans Bell and Green2000; Suan et al. Reference Suan, Pittet, Bour, Mattioli, Duarte and Mailliot2008b; Boulila et al. Reference Boulila, Galbrun, Huret, Hinnov, Rouget, Gardin and Bartolini2014) (Fig. 1). It is marked by a negative carbon isotope excursion (CIE) of around 3–4 per mille in both carbonate and organic carbon substrates (Suan et al. Reference Suan, van de Schootbrugge, Adatte, Fiebig and Oschmann2015), which has been documented in many locations in Europe (Sabatino et al. Reference Sabatino, Neri, Bellanca, Jenkyns, Baudin, Parisi and Masetti2009, Reference Sabatino, Vlahovic, Jenkyns, Scopelliti, Neri, Prtoljan and Velic2013; Kafousia et al. Reference Kafousia, Karakitsios, Jenkyns and Mattioli2011), as well as in NW Africa (Bodin et al. Reference Bodin, Krencker, Kothe, Hoffmann, Mattioli, Heimhofer and Kabiri2016), North and South America (Al-Suwaidi et al. Reference Al-Suwaidi, Angelozzi, Baudin, Damborenea, Hesselbo, Jenkyns, Mancenido and Riccardi2010; Martindale et al. Reference Martindale, Them, Gill, Marroquín and Knoll2017), Siberia (Suan et al. Reference Suan, Nikitenko, Rogov, Baudin, Spangenberg, Knyazev, Glinskikh, Goryacheva, Adatte, Riding, Föllmi, Pittet, Mattioli and Lecuyer2011) and Japan (Izumi et al. Reference Izumi, Miyaji and Tanabe2012). In many localities, laminated black shales were deposited during this time interval with lipid-rich (high hydrogen index) and high total organic carbon (TOC) values and abundant green algal organic matter. Biomarkers indicating photic zone euxinia (Schouten et al. Reference Schouten, Kaam-Peters, van Schoell and Sinninghe Damsté2000; Frimmel et al. Reference Frimmel, Oschmann and Schwark2004; Ruebsam et al. Reference Ruebsam, Muller, Kovacs, Palfy and Schwark2018) provide evidence for strong water column stratification at the zenith of the carbon cycle perturbation (van de Schootbrugge et al. Reference van de Schootbrugge, Bachan, Suan, Richoz and Payne2013).

Fig. 1. Zonal subdivision of the uppermost Pliensbachian and Toarcian successions of the Tethyan and Sub-tethyan, sub-boreal, boreal and Arctic realms, and FO of some important species of the dinoflagellate cysts in the different palaeogeographical realms. Modified after Nikitenko et al. (Reference Nikitenko, Shurygin, Knyazev, Meledina, Dzyuba, Lebedeva, Peshchevitskaya, Glinskikh, Goryacheva and Khafaeva2013b).
In Europe, Toarcian black shales formed in response to the interplay between four main factors with strong feedback links: (1) temperature reconstructions based on belemnite and brachiopod calcite document a substantial (> 7°C) temperature increase of ocean waters at the onset of the Toarcian OAE (Bailey et al. Reference Bailey, Rosenthal, McArthur, van de Schootbrugge and Thirlwall2003; Rosales et al. Reference Rosales, Quesada and Robles2004; van de Schootbrugge et al. Reference van de Schootbrugge, MacArthur, Baily, Rosenthal, Wright and Miller2005; Suan et al. Reference Suan, Mattioli, Pittet, Mailliot and Lecuyer2008a; Korte et al. Reference Korte, Hesselbo, Ullmann, Dietl, Ruhl, Schweigert and Thibault2015), making greenhouse warming a likely contributor to ocean deoxygenation; (2) ocean deoxygenation was regionally exacerbated by elevated rates of marine organic matter production, mainly driven by green algal primary producers, cyanobacteria, and secondary microbial producers, such as sulphur bacteria, leading to bottom water anoxia and photic zone euxinia (Bucefalo Palliani et al. Reference Bucefalo Palliani, Mattioli and Riding2002; van de Schootbrugge et al. Reference van de Schootbrugge, Bachan, Suan, Richoz and Payne2013); (3) greenhouse warming also increased stratification of the water column, leading to extremely slow mixing of deeper waters, further enhancing oxygen depletion (McArthur et al. Reference McArthur, Algeo, van de Schootbrugge, Li and Howarth2008); and (4) circulation was further impeded by elevated run-off from continental landmasses (Cohen et al. Reference Cohen, Coe, Harding and Schwark2004; Dera et al. Reference Dera, Pellenard, Neige, Deconinck, Puceat and Dommergues2009a; Them et al. Reference Them, Gill, Selby, Grocke, Friedman and Owens2017) altering surface water salinity and delivering essential nutrients to fuel primary production (Montero-Serrano et al. Reference Montero-Serrano, Föllmi, Adatte, Spangenberg, Tribovillard, Fantasia and Suan2015; Xu et al. Reference Xu, Ruhl, Jenkyns, Leng, Huggett, Minisini, Ullmann, Riding, Weijers, Storm, Percival, Tosca, Idiz, Tegelaar and Hesselbo2018).
When the geographic distribution of black shales in epicontental NW European marine basins is taken into account, it becomes clear that ocean circulation also played a significant role in driving spatial differences in anoxia (Harazim et al. Reference Harazim, van de Schootbrugge, Sorichter, Fiebig, Weug, Suan and Oschmann2013). In sections close to the palaeo-equator, for example in Portugal (Da Rocha et al. Reference Da Rocha, Mattioli, Duarte, Pittet, Elmi, Mouterde, Cabral, Comas-Rengifo, Gomez, Goy, Hesselbo, Jenkyns, Littler, Mailliot, De Oliveira, Osete, Perilli, Pinto, Ruget and Suan2016) and Morocco (Bodin et al. Reference Bodin, Mattioli, Fröhlich, Marshall, Boutib, Lahsini and Redfern2010), no black shales formed; however, further north in the UK and Germany, TOC values drastically increase and, in some basins, black shale deposition continued throughout the Toarcian Age, well beyond the immediate negative CIE. For example, in the Grands Causses Basin in southern France, sediment deposition continued in anoxic facies following the Schistes Cartons Formation (Harazim et al. Reference Harazim, van de Schootbrugge, Sorichter, Fiebig, Weug, Suan and Oschmann2013), while in Germany upper Toarcian paper shales are largely indistinguishable from those of the lower Toarcian (van de Schootbrugge et al. Reference van de Schootbrugge, Richoz, Pross, Luppold, Hunze, Wonik, Blau, Meister, van der Weijst, Suan, Fraguas, Fiebig, Herrle, Guex, Little, Wignall, Püttmann and Oschmann2018).
Regional differences in the intensity of organic matter deposition as well as the prolongation of anoxia in some parts of the European Epicontinental Seaway (EES) were mainly driven by reorganizations in ocean circulation. Connections with the Arctic Sea through the Viking Corridor and circulation within the Tethys Ocean likely played particularly important roles in oxygenation, stratification and isolation of basins across the EES (Dera & Donnadieu, Reference Dera and Donnadieu2012). However, different authors have arrived at very different conclusions regarding the importance, timing, direction and intensity of flow through the Viking Corridor, with major implications for our understanding of biotic and chemical evolution during the Pliensbachian–Toarcian interval. Prauss & Riegel (Reference Prauss and Riegel1989) were the first to advocate a significant role for the influx of low-salinity Arctic waters to drive density stratification during the early Toarcian Age, using mainly the abundance of prasinophyte green algae in the EES and their presumed cold-water, low-salinity affinity. Bjerrum & Surlyk (Reference Bjerrum and Surlyk2001) used numerical modelling to suggest that flow through the Viking Corridor was mainly directed southwards. Their model was supported by Dera et al. (Reference Dera, Puceat, Pellenard, Neige, Delsate, Joachimski, Reisberg and Martinez2009b) based on sparse Nd-isotope data. In contrast, from a compilation of oxygen isotope data Korte et al. (Reference Korte, Hesselbo, Ullmann, Dietl, Ruhl, Schweigert and Thibault2015) deduced that flow was mainly northwards for the entire duration of the Toarcian Age and that tectonic movements led to a shutdown of warm water flow only during the Aalenian Age, triggering cooling in the northern high latitudes. More recently, Ruvalcaba Baroni et al. (Reference Ruvalcaba Baroni, Pohl, van Helmond, Papadomanolaki, Coe, Cohen, van de Schootbrugge, Donnadieu and Slomp2018) used trace-element data and coupled ocean–atmosphere model runs to argue for strong clockwise currents in the Tethys and complex bathymetry in the EES as important factors in determining regional oxygenation.
In addition to geochemical proxy records, water mass exchange between basins can be studied in great detail using the stratigraphic distribution of nektonic, planktonic and benthic fossil groups such as ammonites, bivalves or microfossils. Here, we examine dinoflagellate cyst records from sections strategically situated at the northern end (Siberia, Russia), and within (offshore Norway) and at the southern end of the Viking Corridor (Yorkshire, UK). Phytoplankton, eukaryotic single-celled algae that float with the currents, are sensitive tracers of water mass conditions, including temperature, salinity and redox state controlling nutrient availability. In general, tolerance of phytoplankton taxa for temperature and salinity variations is larger than for macro-organisms such as cephalopods; phytoplankton therefore respond quicker to opening gateways. As such, palynology provides a powerful tool to trace changes in oceanography, as has been shown for other (oceanic anoxic) events (Vellekoop et al. Reference Vellekoop, Smit, van de Schootbrugge, Weijers, Galeotti, Sinninghe Damsté and Brinkhuis2015; van Helmond et al. Reference van Helmond, Sluijs, Papadomanolaki, Plint, Gröcke, Pearce, Eldrett, Trabucho-Alexandre, Walaszczyk, van de Schootbrugge and Brinkhuis2016). Prauss (Reference Prauss1989), Prauss & Riegel (Reference Prauss and Riegel1989) and Prauss et al. (Reference Prauss, Ligouis, Luterbacher, Tyson and Pearson1991) suggested that Toarcian and Aalenian–Bajocian cyst-producing dinoflagellate diversity in NW Europe was mainly determined by sea-level changes that allowed for exchange between the Arctic and the Tethys. This view has been echoed in later work by Riding, Bucefalo Palliani and Poulsen. However, all these authors were limited by the fact that all the data to support oceanographic models derived from NW Europe. Here, we present for the first time robust stratigraphic dinoflagellate cyst data from both ends of the Viking Corridor.
2. Geological background
To trace ocean circulation between the Arctic Sea and Tethys Ocean through the Viking Corridor (Figs 1, 2) we use the spatial and temporal distribution of Early Jurassic dinoflagellate cysts and present new data for the Kelimyar River S16 and Kelimyar River S5-D1 sections (Fig. 3) in the Olenek River area (Arctic part of Eastern Siberia, Russia; Fig. 1). We compare our data from Siberia with records from within the Viking Corridor from the northern part of the North Sea offshore Norway (Core 34/10-35 from an exploration well in the Gullfaks South Field) using bulk organic carbon isotopes for correlation. Tracing dinoflagellate migrations further south, we use new palynological and carbon isotope data from a key composite section of exposures along the Yorkshire coast (Cleveland Basin, UK; Fig. 1). We compare/integrate our new data with previously published accounts of quantitative and qualitative dinoflagellate records from high- and low-latitude sites.

Fig. 2. Current geography and detailed maps for the investigated locations: (1) Kelimyar River, Siberia, Russia; (2) Gulfaks oil field offshore Norway; and (3) Yorkshire, UK.
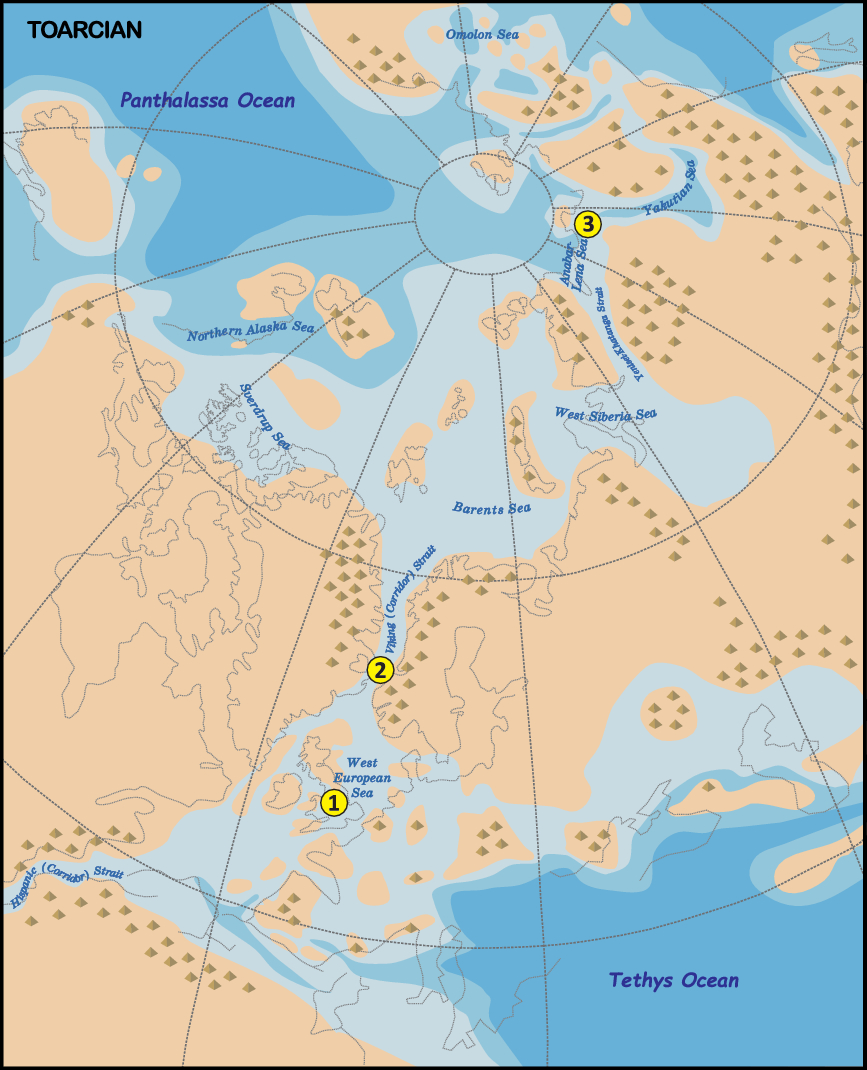
Fig. 3. Palaeogeographic reconstruction for the Toarcian Stage (182 Ma) using G-Plates. Plate tectonic reconstructions after Scotese (Reference Scotese2016) with modifications; Arctic palaeogeography reconstructions after Nikitenko & Mickey (Reference Nikitenko, Mickey, Beaudoin and Head2004) with modifications. 1, Yorkshire Coast, Cleveland Basin (UK); 2, Gulfaks 34/10-35S core (offshore Norway); and 3, Kelimyar River (Russia).
Along the Yorkshire Coast (UK), between the town of Staithes in the north down to the hamlet of Ravenscar in the south, the Lower Jurassic sequence of the Cleveland Basin is exposed in the coastal cliffs, in several partially repetitive outcrops (see Howard, Reference Howard1985; Hesselbo & Jenkyns, Reference Hesselbo, Jenkyns and Taylor1995; Simms et al. Reference Simms, Chidlaw, Morton and Page2004). The Lower Jurassic succession of the Cleveland Basin is dominated by mudrocks. An abundance of ammonites has long enabled this lithostratigraphical framework to be tied in precisely to the ammonite biostratigraphy for the Lower Jurassic sequence.
We have sampled the Pliensbachian–Toarcian succession (Staithes Sandstone, Cleveland Ironstone, Whitby Mudstone and Blea Wyke Sandstone formations) at various locations between Staithes and Runswick Bay and near Kettleness. The upper Toarcian succession was sampled SE of the Peak Fault near Ravenscar. The Staithes Sandstone Formation is approximately 25 m thick, and is dominated by tempestitic sandstones and siltstones. It extends up into the Margaritatus Zone and spans the Capricornus, Figulinum and Stokesi subzones. The Cleveland Ironstone Formation, encompassing the remainder of the Margaritatus Zone and the entire Spinatum Zone, is characterized by silty mudstone coarsening-upward cycles capped by oolitic ironstones. The base of the overlying Whitby Mudstone Formation is taken at a rapid upwards change to mudstone and is coincident with the Pliensbachian–Toarcian boundary (Simms et al. Reference Simms, Chidlaw, Morton and Page2004). The Whitby Mudstone Formation is characterized by a dark, more than 100-m-thick mudstone-dominated succession. It is divided into five members, commencing with the silty mudstones and calcareous or sideritic nodule bands of the Grey Shale Member, passing through the predominantly bituminous shales of the Mulgrave Shale and Alum Shale members, before passing back into silty mudstones of the Peak Mudstone Member and finally the still more silty Fox Cliff Siltstone Member (Simms et al. Reference Simms, Chidlaw, Morton and Page2004).
The Jet Rock contains the negative CIE of the Toarcian Oceanic Anoxic Event (Fig. 4) and has substantially elevated organic carbon contents. Above the Whitby Mudstone Formation, the overlying Blea Wyke Sandstone Formation at the top of the Toarcian Stage, encompasses much of the Dispansum and Pseudoradiosa zones. It is divided into the Grey Sandstone Member, of muddy micaceous siltstone or fine sandstone, and the overlying and somewhat coarser Yellow Sandstone Member. However, across much of the Cleveland Basin the upper part of the Toarcian succession is absent, with the base of the Dogger Formation (Middle Jurassic) resting unconformably upon various levels in the Alum Shale Member. Only to the SE of the Peak Fault, as at Blea Wyke in the Peak Trough, are these higher Toarcian units preserved.

Fig. 4. Lithostratigraphy and geochemical proxy data for the Kelimyar River sections KR-S16 and KR-S5-D1 (modified from Suan et al. Reference Suan, Nikitenko, Rogov, Baudin, Spangenberg, Knyazev, Glinskikh, Goryacheva, Adatte, Riding, Föllmi, Pittet, Mattioli and Lecuyer2011).
From the Cleveland Basin, we analyzed 72 samples for palynology. Because of the lithological distinction among units and the various concretionary marker beds, we were able to accurately correlate the Grey Shales to Jet Rock interval to the bulk-organic carbon isotope curves of Kemp et al. (Reference Kemp, Coe, Cohen and Schwark2005, Reference Kemp, Coe, Cohen and Weedon2011) and Littler et al. (Reference Littler, Hesselbo and Jenkyns2010). We therefore adopt their bulk organic carbon isotope dataset between 210 and 236 m composite position. The remainder of the Pliensbachian–Toarcian carbon isotope is new.
Records from within the Viking Corridor can only be studied through boreholes. Here, we present marine palynological and organic carbon isotope data from Well 34/10-35 (Fig. 5), which was drilled on the Tjalve Terrace SE of the Gullfaks South field in the northern North Sea (61° 4′ 16.17″ N and 2° 19′ 16.77″ E). The well was drilled in a water depth of 135 m and reached a total depth of 4310 m in the lowermost Jurassic Statfjord Group. The main exploration targets were the sandstones of the Middle Jurassic Brent Group and the Pliensbachian–Toarcian Cook Formation, and therefore extensive cores were cut. Cores 1–11 cover the interval between 3931 and 4063.6 m measured depth (MD), and span the upper part of the Burton Formation, the Cook Formation and the Drake Formation. These lithostratigraphic interpretations are after the Norwegian Petroleum Directorate (NPD) Factpages (https://www.npd.no/en/facts/). The top of the investigated section reaches into the Middle Jurassic Brent Group. The lowermost part of the section (4063.6–4048 m MD) is characterized by grey, sandy to silty mudstones ascribed to the Burton Formation. The basal part (up to 4048 m MD) of the overlying Cook Formation is characterized by grey micaceous sandy shales that grade into light yellowish-brown fine carbonate-cemented hard micaceous sandstones (up to 3984 m MD). The transition to the overlying Drake Formation is not recovered by core and is positioned between 3970 and 3983 m MD. The Drake Formation here consists of olive black fissile slightly silty shale. The transition to the Brent Group (Ness Formation) is very sharp and unconformable at 3941.5 m MD. Based on palynostratigraphic data, the Cook Formation is inferred to be of Pliensbachian–Toarcian age (Vollset & Dore, Reference Vollset and Doré1984). Organic carbon isotope data required to constrain the position of the Toarcian CIE in the Cook–Drake sequence of the northern North Sea were previously unavailable. A total of 34 samples was therefore analysed for bulk organic carbon isotopic composition and total organic content. A total of 24 samples was processed and analysed for palynology.

Fig. 5. Range chart and carbon isotope record for the Yorkshire Coast (Cleveland Basin, UK). The section is a composite (see geological background for a description of sampling locations).
The Kelimyar River S5-D1 section was studied by Devyatov et al. (Reference Devyatov, Knyazev and Nikitenko2010) and Nikitenko et al. (Reference Nikitenko, Reolid and Glinskikh2013a) for ammonites and foraminifera, and by Goryacheva (Reference Goryacheva2017) for palynology. The section exposes the subsections Trench 1 and 2, the former being affected by a landslide in its upper part. In this study, the studied samples originate from the lower part of Trench 1 below the inferred landslide. In both sections KR-S16 and KR-S5-D1, the upper Pliensbachian Kyra Formation is dominated by siltstones and sandy siltstones with occasional rounded pebbles and gravels. These sediments were deposited in a shallow shelf setting that experienced episodic erosion during phases of low sea level. Benthic foraminifera indicate that the JF9 Recurvoides taimyrensis foraminiferal Zone (JF9b) is highly condensed and is missing entirely in section KR-S5 (Devyatov et al. Reference Devyatov, Knyazev and Nikitenko2010). In Siberia, this zone corresponds to the base of the Antiquum Zone (equivalent to the European Tenuicostatum Zone; Fig. 6). Accordingly, and considering previous palaeogeographic reconstructions of the Olenek River Basin, the succession exposed in KR-S5-D1 was likely deposited in a more proximal setting than that recorded in KR-S16. In both sections, the overlying lower Toarcian Kurung Member of the Kelimyar Formation is characterized by laminated black shales with elevated TOC values (Suan et al. Reference Suan, Nikitenko, Rogov, Baudin, Spangenberg, Knyazev, Glinskikh, Goryacheva, Adatte, Riding, Föllmi, Pittet, Mattioli and Lecuyer2011; see also new KR-S5-D1 data in Fig. 3) and large concretions. The remainder of the Kelimyar Formation consists of siltstones and silty shales with small concretions and abundant belemnite rostra. Ammonites and benthic foraminifera indicate a late Toarcian age (Devyatov et al. Reference Devyatov, Knyazev and Nikitenko2010; Suan et al. Reference Suan, Nikitenko, Rogov, Baudin, Spangenberg, Knyazev, Glinskikh, Goryacheva, Adatte, Riding, Föllmi, Pittet, Mattioli and Lecuyer2011).

Fig. 6. Range chart and carbon isotope record for the Gulfaks 34/10-35 core.
3. Methods
3.a. Palynology
Palynological processing for samples from Kelimyar River was performed both at the Goethe University Frankfurt (Germany) and Utrecht University (The Netherlands) using similar laboratory protocols for acid digestion, sieving and slide preparation. Samples were dried, and carbonate and silicate minerals were removed by reacting c. 10–20 g of small chips of sediment with concentrated HCl and HF in alternating steps, followed by neutralization with distilled water. To free individual palynomorphs from the large amounts of amorphous organic matter and pyrite that typically dominate Toarcian samples in the Siberian sections, additional treatment with Schultze’s solution (KClO3 + HNO3) was performed. Sieving of samples occurred with a 15 micron mesh sieve. For the Siberian samples, permanent strew mounts were made using ‘glue-for-glas’, which is an ultraviolet (UV) light curing adhesive. The samples from the Cleveland Basin and Well 34/10-35 were prepared at Utrecht University following a comparable protocol. Slides were prepared using the addition of glycerine jelly. For the Cleveland Basin dataset and for Well 34/10-35, UV-reflective microscopy was applied whenever large amounts of amorphous organic matter (AOM) hindered conventional optical analysis. Here, we only present range chart data for dinoflagellate cysts genera and species. Semiquantitative marine and terrestrial palynological records will be discussed in future work.
3.b. Geochemistry
Bulk organic carbon isotope data from Well 34/10-35 and the Cleveland Basin composite section were generated at Iso-Analytical Ltd based in Crewe (UK). Ratios were measured on a continuous-flow isotope ratio mass spectrometer (Europa Scientific 20-20 IRMS) with dual inlet, connected to an elemental analyser (EA-IRMS). Sample powders were pre-treated with 4 M HCl to remove any carbonate phases. Reproducibility, as determined via replicate measurements of 20% of the samples, was ±0.1‰ (1 standard deviation). TOC was analysed both with EA-IRMS and with a LECO-CS230 analyser after removal of carbonate with 4 M HCl. The two methods provided comparable results.
The organic carbon isotope compositions (δ13CTOC) and total organic carbon (TOCcf) contents were measured on 16 samples from Kelimyar River S5-D1 at the Laboratory of Geology of Lyon: Earth, Planets and Environment (LGLTPE, France). About 0.3–0.4 g of bulk rock powder was decarbonated using 1 M hydrochloric acid for 15 min at ambient temperature. The samples were sequentially rinsed four times with deionized water, with 40 min between rinses to allow the samples to settle before being dried in an oven at 50°C. Between 0.5 and 1 mg of decarbonated powder was weighed into tin capsules and placed in a Pyrocube® elemental analyser connected to an Elementar Isoprime® isotope-ratio mass spectrometer in continuous flow. Each analytical run contained four sets of two standards (IAEA CH7 and caseine) to monitor analytical precision and accuracy. Some samples were replicated and the stable isotope results are reported relative to the ‘Vienna Pee Dee belemnite’ (VPDB). The precision was better than 0.15‰ for carbon isotope ratio, and 5% of the reported value for TOC contents of the carbonate-free fraction.
4. Results
4.a. Chemostratigraphy
Pliensbachian and Toarcian organic carbon isotope records have been widely used for correlating sections. Although not without caveats related to changes in organic matter composition, as outlined in Suan et al. (Reference Suan, van de Schootbrugge, Adatte, Fiebig and Oschmann2015) and van de Schootbrugge et al. (Reference van de Schootbrugge, Payne, Tomasovych, Pross, Fiebig, Benbrahim, Föllmi and Quan2008), organic carbon isotope records provide evidence for a prominent negative CIE that has been observed in Toarcian sections around the world (Hesselbo et al. Reference Hesselbo, Grocke, Jenkyns, Bjerrum, Farrimond, Morgans Bell and Green2000, Reference Hesselbo, Jenkyns, Duarte and Oliveira2007; Hesselbo & Pienkowski, Reference Hesselbo and Pienkowski2011). The negative CIE in the Exaratum Subzone is preceded by a smaller negative excursion at the Pliensbachian–Toarcian boundary that is recorded in only a few locations, most likely because of stratigraphic gaps in many records at this level. In the Siberian and Viking Corridor records this Pliensbachian–Toarcian boundary excursion is missing as a result of erosion and condensation (Figs 3, 5). It is most clearly expressed in the Cleveland Basin record as previously reported by Littler et al. (Reference Littler, Hesselbo and Jenkyns2010; see also Fig. 4). The main negative CIE that marks the T- OAE is recorded in all four bulk organic carbon records presented here. As discussed in Suan et al. (Reference Suan, Nikitenko, Rogov, Baudin, Spangenberg, Knyazev, Glinskikh, Goryacheva, Adatte, Riding, Föllmi, Pittet, Mattioli and Lecuyer2011), the early Toarcian negative CIE occurs in the basal part of the Kurung Member in Siberia (Fig. 3). It constitutes a negative shift of 4–5‰ from an upper Pliensbachian baseline of −26‰. Both sections show an abrupt shift that indicates erosion of the lowermost Toarcian. Purely based on lithological thickness, the uppermost Pliensbachian is thinner in the KR-S5-D1 section, whereas the base of the Falciferum Zone, corresponding to the onset of organic-rich sedimentation and anoxia, is more expanded. In both sections, the isotope record does not return to pre-CIE or more positive values, which is likely an artefact of organic matter sourcing.
The Gullfaks 34/10-35 core record shows a clear negative CIE starting at 4025 m MD with values decreasing from −26 to −29‰ (Fig. 5). The CIE spans a broad interval and values remain low at c. −29‰ until 3985 m MD. Values thereafter increase and reach −24‰ at the erosional contact between the Drake Formation and the Ness Formation. The negative CIE in the Yorkshire record has been well studied, and the record presented here reproduces these older datasets in every detail. Values remain rather low at c. −28‰ for much of the Falciferum and Bifrons zones. A modest positive trend of +2‰ is observed for the upper Toarcian Stage.
4.b. Palynostratigraphy
4.b.1. Cleveland Basin
Dinoflagellate cysts are absent from the upper Pliensbachian Staithes Sandstone and base of the Cleveland Ironstone Formation (Fig. 4). This is ascribed to the paralic depositional nature of the succession. The first dinoflagellate cyst species to appear at the top of the Margaritatus Zone is the marker species Luehndea spinosa, which is widespread in NW Europe and Tethyan sections and straddles the Pliensbachian–Toarcian boundary. Its extinction has been used to mark the onset of the T-OAE in Tethyan sections (Bucefalo Palliani & Riding, Reference Bucefalo Palliani and Riding1999; Bucefalo Palliani et al. Reference Bucefalo Palliani, Mattioli and Riding2002). The appearance of L. spinosa is followed by a veritable regional increase in diversity during the Spinatum Zone with the appearance of key taxa representing several of the characteristic Early Jurassic dinoflagellate lineages, including Nannoceratopsis deflandrei subsp. senex, N. deflandrei subsp. gracilis, Scriniocassis weberi, Maturodinium inornatum and Valvaeodinium armatum. The Pliensbachian–Toarcian boundary shows the first appearance of further key taxa, including Mancodinium semitabulatum and Limbicysta bjaerkei. The lower Toarcian Tenuicostatum Zone witnessed the sustained presence of all these taxa as well as the first appearance of Wallodinium cylindricum. The lower part of the Falciferum Zone, coincident with the negative CIE, shows a strong increase in the abundance of amorphous organic matter. The dominant palynomorphs here are Halosphaeropsis liassica, small leiospheres most likely representing early maturation stages in the life cycle of prasinophyte green algae (van de Schootbrugge et al. Reference van de Schootbrugge, Bachan, Suan, Richoz and Payne2013). The base of the Mulgrave Shale Member, showing the negative CIE (Exaratum Subzone), is characterized by a marked decrease in dinoflagellate cyst abundance and diversity. All encountered taxa, except for Wallodinium cylindricum and Nannoceratopsis triceras, range through and reappear after the interval with the negative CIE. However, both W. cylindricum and N. triceras are extremely rare in the section, which could account for their disappearance.
The transition from the Falciferum to the Bifrons Zone witnessed a further increase in diversity with the first appearance of members of the Parvocysta–Phallocysta suite. The first true member of the Parvocystaceae to appear is Parvocysta bullula. The boundary between the Falciferum and Bifrons zones also coincides with a lithological change from the paper shales of the Mulgrave Shale Member to the less organic-rich Alum Shale Member. The abundance of Halosphaeropsis also decreases across this boundary. In the middle part of the Bifrons Zone the diagnostic species Susadinium scrofoides and Phallocysta eumekes have their first occurrence (FO). At the boundary between the lower and upper Toarcian Stage, Scriniocassis priscum joins some of the longer-ranging taxa such Mancodinium semitabulatum and Nannoceratopsis gracilis.
Our data are complementary to previous work by Riding (Reference Riding1984) on the Blea Wyke area around Ravenscar (Yorkshire, UK) and by Bucefalo Palliani & Riding (Reference Bucefalo Palliani and Riding2000) on coastal outcrops between Staithes and Robin Hood’s Bay. The study by Bucefalo Palliani & Riding (Reference Bucefalo Palliani and Riding2000) was mainly directed at the Sinemurian and upper Pliensbachian stages with few samples from the Toarcian Whitby Mudstone Formation. Riding (Reference Riding1984) focused mostly on the upper Toarcian Variabilis to Aalenian Opalinum zones, recognizing many of the same taxa as presented here. Based on all three studies, the following stratigraphic ranges can be precisely delineated. In the Cleveland Basin, the first species of Nannoceratopsis and Mancodinium semitabulatum appear in the lower Pliensbachian (Ibex Zone) succession, ranging all the way to the base of the Aalenian Stage. The dinoflagellate cyst assemblages observed from the lower Toarcian Stage are dominated by Valvaeodinium and Nannoceratopsis with sparse Mendicodinium, Mancodinium and Luehndea. Scriniocassis weberi has a continuous range from the Spinatum Zone to the lower Aalenian strata based on the combined records from Riding (Reference Riding1984) and this study. Phallocysta eumekes ranges from the middle Bifrons Zone to the Thouarsense Zone. The range of Scriniocassis priscum is slightly more extensive based on the work by Riding (Reference Riding1984), occurring as early as in the Bifrons Zone.
The first specimens of Parvocysta in the record presented by Riding (Reference Riding1984) occur within the upper Toarcian Variabilis Zone with the first occurrence of Parvocysta nasuta, which is slightly above the first occurrence of P. bullula noted in this study. Facetodinium inflatum, not recognized in this study, first occurs within the upper Toarcian Thouarsense Zone in the study by Riding (Reference Riding1984). Limbicysta bjaerkei, recognized by Riding (Reference Riding1984) as Parvocysta cf. barbata, has a continuous range from the upper Pliensbachian to the Aalenian successions. Wallodinium cylindricum has a range from the lower Toarcian Tenuicostatum to upper Toarcian Levesqui Zone, but disappears for most of the middle Toarcian Stage. Finally, Riding (Reference Riding1984) encountered Escharisphaeridia sp. in many upper Toarcian samples starting in the Variabilis Zone. Similar specimens were also encountered by us, but they were difficult to quantify. More detailed analyses with regards to this clade are ongoing. This genus shows typical gonyalaucoid paratabulation and archeopyle styles and can be seen as an early representative of the Gonyalaucales, a major Mesozoic lineage of dinoflagellates that rose to prominence during the Bajocian (Wiggan et al. Reference Wiggan, Riding, Fensome and Mattioli2018).
4.b.2. Gullfaks South Field core 34/10-35
Palynological assemblages for Core 34/10-35 for the upper Pliensbachian Stage from the Burton Formation and Toarcian Drake and Cook formations (Fig. 5) are similar to those from Yorkshire, but differ in several important details. The ranges of Nannoceratopsis gracilis and Mancodinium semitabulatum are in accordance with many other records from NW Europe. The key species Luehndea spinosa ranges across the Pliensbachian–Toarcian boundary and disappears at the height of the negative CIE within the Cook Formation. The range of Valvaeodinium is comparable to L. spinosa; there is therefore no apparent ‘dinoflagellate cyst black-out’. Another striking difference with the Cleveland Basin record is the early inception of gonyalaucoid cysts attributable to the genera Batiacasphaera and Dissiliodinium. Both cyst types occur in the Pliensbachian succession and range through the interval that contains the negative CIE. Their presence prior to the negative CIE within the Viking Corridor suggests a much earlier inception of these gonyalaucoid cysts when compared with the British records. Third, and perhaps most important, is the first inception of members of the Parvocysta-suite coincident with the negative CIE. Parvocysta bullula and P. nasuta have their first appearances within the middle part of the Cook Formation, which, based on the C-isotope stratigraphy, is equivalent to the lower Toarcian Stage.
4.b.3. Kelimyar River S16
The Kelimyar River (KR) Section S16 shows distinct palynological assemblages for Pliensbachian and Toarcian formations (Figs 7, 8). Within the KR-S16 section, the lower part of the upper Pliensbachian Kyra Formation (Viligaensis Zone) is characterized by abundant terrestrial organic matter. Dinoflagellate cysts in this interval are sparse and dominated by Nannoceratopsis senex and N. gracilis and rare Mancodinium semitabulatum. Other marine elements include rare prasinophyte phycomata and acritarchs. The uppermost part of the Kyra Formation shows a clear increase in marine influence with the appearance of Limbicysta bjaerkei, and especially in sample KR-S16(09) where Valvaeodinium armatum, V. stipulatum, V. cavum and Batiacasphaera sp. have their first appearances.

Fig. 7. Range chart for the Kelimyar River S16 section showing selected dinoflagellate species. A number of dinoflagellate cysts are undescribed and not included in the chart.

Fig. 8. Selected Pliensbachian and Toarcian dinoflagellate cyst species from Kelimyar River section S16 (all scale bars, 20 μm). (a) Limbicysta bjaerkei: sample S16-07 from the Viligaensis Zone, upper Pliensbachian. Note the extremely bifurcated processes, illustrating intraspecific variability. (b) Limbicysta bjaerkei: sample S16-80 (Kelimyar Formation), upper Toarcian. Note the generally more robust shape and processes compared with the Pliensbachian specimen. (c) Parvocysta cracens: sample S16-71 from the upper Toarcian Kelimyar Formation. (d) Susadinium scrofoides: sample S16-48 from the Commune Zone (lower Toarcian, Kelimyar Formation). (e) Parvocysta bullula: sample S16-60 from the Commune Zone (lower Toarcian, Kelimyar Formation). (f) Parvocysta tricornuta: sample S16-80 from the upper Toarcian (Kelimyar Formation). (g) Batiacasphaera sp. A: sample S16-80 from the upper Toarcian (Kelimyar Formation). (h) Batiacasphaera sp. B: sample S16-27 from the Falciferum Zone (lower Toarcian, Kelimyar Formation). (i) Dissiliodinium sp.: sample S16-30 from the Falciferum Zone (lower Toarcian, Kelimyar Formation). (j) Phallocysta eumekes: sample S16-60 from the Commune Zone (lower Toarcian, Kelimyar River). (k) Mancodinium semitabulatum: sample S16-09 from the Viligaensis Zone (Kyra Formation, upper Pliensbachian) (l) Mancodinium semitabulatum: sample S16-30 from the Falciferum Zone (lower Toarcian, Kelimyar Formation). (m) Valvaeodinium aquilonium: sample S16-49 from the Commune Zone (lower Toarcian, Kelimyar Formation). (n) Valvaeodinium cavum: sample S16-21 from the Falciferum Zone (lower Toarcian, Kelimyar River).
The transition to the lower Toarcian Kurung Member of the Toarcian Kelimyar Formation shows a dramatic increase in the amount of marine palynomorphs with abundant remains of acritarchs, dinoflagellate cysts and prasinophytes. The lowermost Toarcian Antiquum Zone is highly condensed and only represented by sample KR-S16(10). However, this sample shows a clear increase in dinoflagellate diversity. Nannoceratopsis deflandrei subsp. senex, Mancodinium semitabulatum and Limbicysta bjaerkei continue to be present. A number of genera and species have a first occurrence in this sample (see Fig. 5), including Valvaeodinium stipulatum, Comparodinium punctatum, Valvaeodinium armatum and Batiacasphaera sp. Members of the Parvocysta–Phallocysta suite might be present, but the preservation is not good enough in this sample to be absolutely sure and their identification has to await further analysis. Sample KR-S16(10) shows a brief decline in diversity, but many of the species (M. semitabulatum, N. senex and M. inornatum) that were present below continue within this sample and beyond. Sample KR-S16(12) is the only sample in this set of 33 samples that is devoid of any structured palynomorphs, which is likely a taphonomic problem. Curiously enough, this sample, with the most negative C-isotope value within the Toarcian negative CIE, is entirely dominated by phytoclasts.
Sample KR-S16(15) coincides with the returning limb of the negative CIE within the Falciferum Zone. This sample shows a marked increase in diversity. Many of the species present prior to the negative CIE continue. In addition, this sample marks the first appearance of Scriniocassis weberi, Phallocysta eumekes, Parvocysta bullula and dinoflagellate cysts attributable to the genus Dissiliodinium. Afterwards, diversity continues to increase gradually up until the top of the Commune Zone (sample KR-S16(60)) with the addition of Susadinium scrofoides, Wallodinium cylindricum, Parvocysta nasuta and Maturodinium inornatum. In this interval we also observed several species of Nannoceratopsis deflandrei subsp. senex other than N. senex and N. gracilis, including N. triangulata, N. plegas and N. magnicornus. Diversity decreases again in the Braunianus Zone, which is likely an artefact of changes in lithology and decreased preservation. Parvocysta cracens occurs briefly around the lower–upper Toarcian boundary, and perhaps this species is a good marker for this boundary in high-latitude settings. The uppermost sample, KR-S16(80) from the upper Toarcian Stage, shows the appearance of several additional species, including Fromea tornatilis and Dodekovia pseudochytroides, as well as Parvocysta tricornuta. Although Fromea tornatilis is not generally accepted as a dinoflagellate cyst, the first two species were previously only known from the Aalenian–Bajocian stages of NW Europe; the latter occurs in the upper Toarcian Hebrides Basin (Scotland; Riding et al. Reference Riding, Walton and Shaw1991). Overall, the Kelimyar River S16 record does show the much earlier inception of the Parvocysta–Phallocysta suite, with many key species appearing during the early Toarcian Age.
4.b.4. Kelimyar River S5-D1
Although very much condensed, this section is extremely important for examining the inception of the Parvocysta–Phallocysta suite during the early Toarcian Age. The 10 samples investigated are from the very top part of the Kyra Formation (sample KR-S5-D1(05)) and the lowermost 1.5 m of the Kelimyar Formation (Kurung Member) that shows the negative CIE (Fig. 9). Sample S5(05) shows moderately abundant Valveaeodinium stipulatum in addition to other more poorly preserved specimens of Valvaeodinium sp. With the onset of the Toarcian negative CIE, we observe a dramatic rise in species diversity with the first appearance datums (FADs) of Valvaeodinium aquilonium, Susadinium scrofoides, Wallodinium laganum, Dodekovia pseudochytroides, Batiacasphaera sp., Phallocysta elongata and Mancodinium semitabulatum (see also Fig. 10). At the top of the black shale at the base of the Kurung Member more species appear, including Fromea tornatilis, Moesiodinium raileanui, Phallocysta eumekes and Parvocysta bullula. Sample KR-S5-D1(11) contains several species of Parvocysta, including P. contracta, P. nasuta and Facetodinium inflatum. Surprisingly, Nannoceratopsis species are largely missing, except for higher up in samples KR-S5-D1(14) to (18). These latter samples contain less dinoflagellate cysts, but instead contain abundant amorphous organic matter.

Fig. 9. Range chart for Kelimyar River section S5-D1 with selected dinoflagellate species. A number of species are undescribed and not included in this chart.

Fig. 10. Selected Pliensbachian and Toarcian dinoflagellate cyst species from Kelimyar River section S5-D1 (all scale bars, 20 μm). (a) Valvaeodinium stipulatum: sample S5-05 from the upper Pliensbachian (Kyra Formation). (b) Phallocysta elongate: sample S5-07 from the base of the Kurung Member (lower Toarcian). (c) Phallocysta eumekes: sample S5-10 from the base of the Kurung Member (lower Toarcian). (d) Fromea tornatilis: sample S5-10 from the base of the Kurung Member (lower Toarcian). (e) Facetodinium inflatum: sample S5-09 from the base of the Kurung Member (lower Toarcian). (f) Parvocysta bullula: sample S5-09 from the base of the Kurung Member (lower Toarcian). (g) Parvocysta nasuta: sample S5-11 from the Kurung Member (lower Toarcian). (h) Moesiodinium raileanui: sample S5-10 from the base of the Kurung Member (lower Toarcian). (i) Parvocysta nasuta: sample S5-11 from the Kurung Member (lower Toarcian). (j) Parvocysta contracta: sample S5-11 from the Kurung Member (lower Toarcian). (k) Susadinium scrofoides: sample S5-11 from the Kurung Member (lower Toarcian). (l) Moesiodinium raileanui: sample S5-07 from the base of the Kurung Member (lower Toarcian). (m) Dodekovia pseudochytroides: sample S5-07 from the base of the Kurung Member (lower Toarcian). (n) Batiacasphaera sp. A: sample S5-07 from the base of the Kurung Member (lower Toarcian). (o) Valvaeodinium aquilonium: sample S5-07 from the base of the Kurung Member (lower Toarcian). (p) Wallodinium laganum: sample S5-07 from the base of the Kurung Member (lower Toarcian).
Sections in the Arctic part of the Eastern Siberia Basin, especially in the Anabar and Olenek river deltas, have been investigated previously for palynology and palynostratigraphy by various Russian workers. An enormous body of work was compiled, summarized and complemented by additional analyses in Riding et al. (Reference Riding, Fedorova and Ilyina1999). However, analysis of outcrops along the Kelimyar River was only represented by a single sample (NS53; Riding et al. Reference Riding, Fedorova and Ilyina1999) from the lowermost upper Toarcian strata. That sample was overwhelmingly dominated by several species of Nannoceratopsis and Phallocysta eumekes, in addition to rare Parvocysta cracens, Susadinium scrofoides and Valvaeodinium aquilonium (Riding et al. Reference Riding, Fedorova and Ilyina1999). A higher-resolution study was carried out on sections along the Anabar River spanning the upper Pliensbachian to lower Toarcian successions (two sections, approximately 40 samples; Riding et al. Reference Riding, Fedorova and Ilyina1999). In those sections, the upper Pliensbachian Viligaensis Zone produced sparse assemblages dominated by Nannoceratopsis deflandrei subsp. anabarensis and N. deflandrei subsp. senex. These two subspecies of N. deflandrei also dominate the lower Toarcian Propinquum (=Antiquum), Falciferum and Commune zones. In addition, Riding et al. (Reference Riding, Fedorova and Ilyina1999) mention Mancodinium semitabulatum and N. gracilis as dominant taxa in the lower Toarcian Stage. All common members of the Phallocysta–Parvocysta suite are consistently observed in the upper Toarcian strata by Riding et al. (Reference Riding, Fedorova and Ilyina1999). Species of Valvaeodinium, which are already clearly present within the uppermost Pliensbachian and lowermost Toarcian stages, were only observed within the upper Toarcian Stage in the compilation presented by Riding et al. (Reference Riding, Fedorova and Ilyina1999). The study by Goryacheva (Reference Goryacheva2017), who worked essentially on the same sample set from sections KR-S16 and KR-S5-D1, produced slightly different results from those presented in Riding et al. (Reference Riding, Fedorova and Ilyina1999). Most notably, Goryacheva (Reference Goryacheva2017) documents the presence of Phallocysta eumekes and several other “typical” upper Toarcian NW European species, such as Fromea, already in the lower Toarcian Kurung Member.
The striking differences between the records from Siberia presented by Riding et al. (Reference Riding, Fedorova and Ilyina1999) and Goryacheva (Reference Goryacheva2017), and between these records and those presented here, could be due to a number of reasons. Although the study by Riding et al. (Reference Riding, Fedorova and Ilyina1999) was generally of lower resolution, the authors did look at a much greater number of sections, so we would expect them to find a similar overall diversity. Taphonomy and regional palaeo-environmental settings could also be issues as most dinoflagellate cysts are extremely thin-walled and preservation, especially in intervals rich in amorphous organic matter, is poorer. Sample location could also be an issue; our study suggests that KR-S5-D1 contains a higher diversity than section KR-S16. The two sections are more than 60 km apart, so the possibility of regional differences in palaeodepths is plausible. The KR-S16 section shows a predominance of Nannoceratopsis species, which seem to be mostly absent from the KR-S5-D1 section. Other striking differences include the abundance and continued presence within the Toarcian Stage of specimens grouped under Batiacasphaera in the KR-S16 section and the diverse and abundant presence of members of the Parvocysta-suite in the lowermost Toarcian strata in the KR-S5-D1 section. These are likely due to regional differences in bathymetry. As the study by Goryacheva (Reference Goryacheva2017) was carried out on the same sample set, it seems that different methods of preparation of samples could be an issue. Goryacheva (Reference Goryacheva2017) mentions the use of heavy liquid to separate minerals from palynomorphs; this technique can lead to loss of small palynomorphs that have pyrite infilling.
5. Discussion
5.a. High-latitude dinoflagellate diversity
The high latitudes have played a pivotal role in understanding the evolution of cyst-producing dinoflagellates (Riding & Ioannides, Reference Riding and Ioannides1996; Mangerud et al. Reference Mangerud, Paterson and Riding2019). Sections in Arctic Canada have yielded some of the oldest and also most diverse dinoflagellate cyst assemblages dating back to the Late Triassic Period (Wiggins, Reference Wiggins1973, Reference Wiggins1978; Bujak & Fisher, Reference Bujak and Fisher1976). Work on phosphorite nodules from the Toarcian Brentskardhaugen Bed on Spitsbergen produced the earliest evidence for the previously unknown Parvocysta suite (Bjaerke, Reference Bjaerke1980). Work by Davies (Reference Davies1983) on a dinoflagellate cyst zonation for the Sverdrup Basin of Arctic Canada showed the presence of a diverse algal community with at least 18 Toarcian species. Prauss (Reference Prauss1989) speculated that early dinoflagellate cyst diversity was higher in the northern high latitudes as many species tend to have earlier first occurrences there when compared with low latitudes. A northern high-latitude origin for the Parvocysta–Phallocysta suite was suggested by Feist-Burkhardt & Wille (Reference Feist-Burkhardt and Wille1992) and Riding & Ioannides (Reference Riding and Ioannides1996) based on palynological records from southern Germany and the UK, respectively. However, precise evidence for the high-latitude origin of the Parvocysta–Phallocysta suite has been generally lacking because of a dearth in studies on systematically sampled sections.
Abundant and diverse lower Toarcian Falciferum Zone dinoflagellate cyst assemblages in the Kelimyar River sections are both interesting and at odds with records of similar age from NW Europe and the Mediterranean area, such as in Germany, the UK and the Lusitanian Basin (Portugal). For example, in Portugal only two genera (Nannoceratopsis and Mancodinium) are present across the T-OAE (Correia et al. Reference Correia, Riding, Duarte, Fernandes and Pereira2017), which is unlikely solely related to taphonomic issues. Further, the Phallocysta–Parvocysta suite is entirely missing from many Tethyan sections (Davies, Reference Davies1985; Poulsen & Riding, Reference Poulsen, Riding and Ineson2003; Riding & Ioannides, Reference Riding and Ioannides1996). Across NW Europe, organic-matter-rich shales of early Toarcian age are characterized by low-diversity dinoflagellate cyst assemblages; in particular, sections that contain extensive laminated black shales (Posidonia shales) are normally devoid of dinoflagellate cysts. In southern Germany, the Unterer and Oberer Schiefer (Lias Epsilon), equivalent to the Falciferum Zone, only contain Nannoceratopsis deflandrei subsp. gracilis, Comparodinium punctatum and C. lineatum (Wille, Reference Wille1982).
Palynological studies carried out in Italy, Germany and the UK provide strong evidence for a rapid spread of the Parvocysta–Phallocysta suite across Europe during late Toarcian time. In Germany, Wille (Reference Wille1982) described Parvocysta nasuta from the Fibulatum Subzone (Bifrons Zone). In his very extensive monograph on dinoflagellate taxonomy and diversity from northern Germany, Prauss (Reference Prauss1989) documented in great detail the late Toarcian diversification of the Parvocysta–Phallocysta suite. In a review of dinoflagellate cyst biostratigraphy for the Danish Sub-basin, Poulsen (Reference Poulsen1992) introduced a “Parvocysta Subzone” based on the first and last occurrences of a whole suite of Parvocysta species, including P. barbata, P. bjaerkei (=Limbicysta bjaerkei), P. bullula, P. cracens, P. nasuta, Susadinium faustum and S. scrofoides. The age of this subzone is early middle Toarcian (Bifrons Zone) to Aalenian. Work by Bucefalo Palliani et al. (Reference Bucefalo Palliani, Cirilli and Mattioli1998) on the upper Toarcian – Aalenian Wittnau core from Germany showed a maximum diversity for the Parvocysta group within the uppermost Toarcian strata. Feist-Burkhardt & Pross (Reference Feist-Burkhardt and Pross2010) also showed high abundances and diversity of the Parvocysta–Phallocysta suite in the uppermost Toarcian and basal Aalenian successions in the Hausen core from southern Germany. Whereas records from South America tend to lack independent age control, the first members of the Parvocysta–Phallocysta suite appear in the uppermost Toarcian Neuquen Basin in Argentina (Zavattieri et al. Reference Zavattieri, Rosenfeld and Volkheimer2008), which could be taken as evidence for a progressive migration from northern high latitudes to southern low latitudes during the course of the Toarcian Age.
The early Toarcian disappearance of dinoflagellate cysts following a late Pliensbachian radiation has been described as a “dinoflagellate cyst black-out” and is widely seen as a result of the inimical conditions within the water column coinciding with the Toarcian Oceanic Anoxic Event (Bucefalo Palliani et al. Reference Bucefalo Palliani, Cirilli and Mattioli1998). Indeed, one of the most common and abundant late Pliensbachian – earliest Toarcian species, Luehndea spinosa, appears to have gone extinct across northern Europe with the onset of black shale deposition (Bucefalo Palliani & Riding, Reference Bucefalo Palliani and Riding1999). The Siberian and Viking Corridor records paint a completely different picture, with numerous dinoflagellate species present at the height of the Toarcian OAE. Instead of being a “dinoflagellate cyst black-out”, the Toarcian OAE interval in northern high latitudes was an interval of rapid diversification with high diversity and abundance. Moreover, early inceptions of the Parvocysta–Phallocysta suite in Siberia were accompanied by the early appearance of members of the Gonyaulaucales, including many undescribed specimens assigned to Escharisphaeridia, Batiacasphaera and Dissiliodinium. These genera typically appear during latest Toarcian – Aalenian time, while several other genera such as Fromea are characteristic of the Aalenian–Bajocian interval. From this, we conclude that the high northern latitudes likely functioned as a cradle for dinoflagellate evolution. The enormous diversity recorded in the KR-S5-D1 section also suggests that this evolution was ongoing. To determine whether it was triggered by carbon injection and enhanced greenhouse warming needs further work, but it could prove to be a pivotal example of the link between greenhouse warming and changes in ecosystem structure and evolution in high-latitude settings.
5.b. Arctic connectivity and circulation through the Viking Corridor
The presence of a diverse lower Toarcian (Falciferum Zone) assemblage of cyst-producing dinoflagellates in Siberia and the Viking Corridor, particularly the presence of the Parvocysta–Phallocysta suite, has implications for our understanding of Early Jurassic oceanography. The sudden inception of the Parvocysta–Phallocysta suite in NW Europe during the late early Toarcian Age (Bifrons Zone) and its further diversification during the late Toarcian Age indicates that an important connection existed between the Arctic Sea and the Tethys Ocean, allowing these phytoplankton taxa to migrate southwards. Our data strongly point towards the establishment of a S-directed circulation through the Viking Corridor at the Falciferum–Bifrons transition. Since some homogenization of dinoflagellate communities occurred prior to the Toarcian OAE during the late Pliensbachian Age, with both the Arctic and Tethys regions showing communities dominated by members of Valvaeodiniaceae and Nannoceratopsiaceae and the cosmopolitan Mancodinium, passages were likely open during the entire Pliensbachian–Toarcian interval.
The delayed arrival of the Parvocysta–Phallocysta suite in NW European basins during the Bifrons Zone relative to the spread of Nannoceratopsis and Valvaeodinium species suggests that some physical or chemical barrier existed that inhibited the Parvocysta–Phallocysta suite to migrate southwards. The widespread genus Nannoceratopsis was likely adapted to coastal habitats and low-salinity, perhaps even brackish, waters. In contrast, Parvocysta–Phallocysta taxa that dominate the lower Toarcian Stage in Siberia are considered here to have been open-marine taxa. Hence, the arrival of the Parvocysta–Phallocysta suite in NW Europe was either driven by eustatic sea-level rise or by tectonic subsidence in the Viking Corridor allowing taxa to spread through the gateway. Indeed, sea-level reconstructions for NW Europe indicate a lowstand during earliest Toarcian time and highstands for the Falciferum–Bifrons transition. In Germany, this level is marked by the Monotis Beds or “Inoceramenbank”, strongly condensed beds rich in bivalves marking a maximum flooding surface (Röhl et al. Reference Röhl, Schmid-Röhl, Oschmann, Frimmel and Schwark2001; Rohl & Schmid-Röhl, Reference Röhl, Schmid-Röhl and Harris2005).
Surface water connection through the Viking Corridor played a crucial role in determining oxygenation of basins at the southern end of the strait across the EES as suggested and reviewed extensively by Korte et al. (Reference Korte, Hesselbo, Ullmann, Dietl, Ruhl, Schweigert and Thibault2015). This flow was likely controlled by tectonic movements modulated by changes in eustatic sea level and perhaps autocyclic infilling. Sedimentary successions within the Viking Corridor are a key piece of the puzzle and inform us of water depth in the corridor. In the Viking Graben area of the northern North Sea (Fig. 2), Lower Jurassic sediments conformably overlie Triassic strata. Upper Triassic – lowermost (Hettangian) Jurassic strata are characterized by the massive deltaic sandstones of the Statfjord Group (Lervik, Reference Lervik2006). The remainder of the Lower Jurassic succession (Sinemurian – base Aalenian) comprises the Dunlin Group, which is divided into (from base to top) the Amundsen, Johansen, Burton, Cook and Drake formations (Vollset & Dore, Reference Vollset and Doré1984). The Amundsen and Burton formations represent fine-grained paralic estuarine to proximal marine shelfal mudstones, whereas the Johansen and Cook formations represent coarser-grained sandstone end-members.
The coarse clastic material of the Cook Formation was sourced from the eastern basin margin and prograded westwards (Chamock et al. Reference Chamock, Kristiansen, Ryseth and Fenton2001; Husmo et al. Reference Husmo, Hamar, Høiland, Johannessen, Rømuld, Spencer, Bathurst, Evans, Graham, Armour and Bathurst2003). Hence, the Cook Formation was deposited at a time when shallow-marine waters had transgressed over parts of the deltaic to paralic Triassic and Lower Jurassic strata (Steel, Reference Steel1993) and formed a very shallow and narrow seaway, essentially bounded by the Shetland Platform in the west and the Fennoscandian hinterland in the east (Husmo et al. Reference Husmo, Hamar, Høiland, Johannessen, Rømuld, Spencer, Bathurst, Evans, Graham, Armour and Bathurst2003). The Cook Formation can be divided into four to five distinct sandy units, with regressive–transgressive segments that can be traced straightforwardly on petrophysical logs (Chamock et al. Reference Chamock, Kristiansen, Ryseth and Fenton2001). As a consequence, the upper part of the Dunlin Group is often interpreted in terms of alternations between sandy (Cook Formation) and mudstone (Drake Formation) facies. Above the fourth and uppermost Cook Formation sandstone, the widespread, conspicuous, dark, episodically calcareous Drake Formation claystone unit occurs (Chamock et al. Reference Chamock, Kristiansen, Ryseth and Fenton2001). The latter is interpreted to mark progressive deepening of the Viking Corridor. Based on our C-isotope correlations, this deepening occurred during the Bifrons Zone, at the time when Arctic dinoflagellates appeared in NW Europe and when conditions changed from euxinic to anoxic.
Interestingly, the Parvocysta–Phallocysta suite disappeared from NW European basins during the Aalenian Age (Prauss, Reference Prauss1989) and their range tops make good stratigraphic markers (Feist-Burkhardt & Pross, Reference Feist-Burkhardt and Pross2010). The Aalenian Stage was marked by thermal doming in the North Sea region effectively interrupting southwards flow through the Viking Corridor. Within the Viking Corridor the Brent Group marks the arrival of shallow-marine deltaic deposits that unconformably overly the Cook and Drake formations. Reorganization of circulation in the northern North Sea could explain the cooling that is observed in belemnite oxygen isotope records from the Hebrides Basin in Scotland (Korte et al. Reference Korte, Hesselbo, Ullmann, Dietl, Ruhl, Schweigert and Thibault2015). The disappearance of the Parvocysta–Phallocysta suite may be linked to interruption of flow through the Viking Corridor. Whether these dinoflagellates continued to thrive in high northern latitudes remains to be determined. Understanding their evolutionary trajectories in the Arctic will likely inform us of the cause of their demise.
5.c. Implications for Toarcian palaeoceanographic models
Our new data have clear implications for palaeoceanographic models that have been invoked to explain salinity stratification within the EES leading to the T-OAE. The influx of low-salinity Arctic waters through the Viking Corridor can be ruled out as a driver of stratification and anoxia (Prauss & Riegel, Reference Prauss and Riegel1989; Prauss et al. Reference Prauss, Ligouis, Luterbacher, Tyson and Pearson1991), since these waters arrived across the transition from the Falciferum to Bifrons zones, well after the onset of anoxia in NW Europe. Our results are fully incompatible with a recently proposed scenario in which melting ice caps that had supposedly developed during the late Pliensbachian Age drove a sea-level rise of 100 m and led to low-salinity water influx at the onset of the T-OAE (Ruebsam et al. Reference Ruebsam, Mayer and Schwark2019). Instead, stratification and poor mixing of basins in the EES and along the western Tethys margin can be fully explained by increased run-off from Laurasia under enhanced greenhouse climate conditions, combined with restriction of basins (McArthur et al. Reference McArthur, Algeo, van de Schootbrugge, Li and Howarth2008) due to sea-level changes and circulation in the Tethys Ocean (Ruvalcaba Baroni et al. Reference Ruvalcaba Baroni, Pohl, van Helmond, Papadomanolaki, Coe, Cohen, van de Schootbrugge, Donnadieu and Slomp2018). Enhanced run-off from Laurasia is supported by clay mineral data from across the EES that shows elevated chemical weathering on the continents (Cohen et al. Reference Cohen, Coe, Harding and Schwark2004) and the influx of kaolinite (Raucsik & Varga, Reference Raucsik and Varga2008; Dera et al. Reference Dera, Pellenard, Neige, Deconinck, Puceat and Dommergues2009a), a clay mineral that is widely understood to form under conditions of extreme humidity.
Reconstructions of Jurassic circulation between the Arctic Sea and Tethys Ocean have relied on geochemical proxies, faunal migrations and output from coupled ocean–atmosphere models. Work on ammonite and foraminifera diversity suggests that the Viking Corridor served as a major gateway for faunal exchange between high- and low-latitude communities (Nikitenko & Mickey, Reference Nikitenko, Mickey, Beaudoin and Head2004; Zakharov et al. Reference Zakharov, Shurygin, Il’ina and Nikitenko2006). Ammonite faunas show signs of maximum separation during the Late Pliensbachian, but faunas became entirely homogenized during the Falciferum Zone with Tethyan ammonite families such as the Dactylioceratinae migrating northward into Arctic basins (Dera et al. Reference Dera, Neige, Dommergues and Brayard2011). Foraminifera, ostracodes and bivalves show a major turnover across the Pliensbachian–Toarcian boundary in Siberia, which has been explained by migration through the Viking Corridor due to changes in sea level and climate (Nikitenko & Shurygin, Reference Nikitenko, Shurygin, Thurston and Fujita1994; Nikitenko & Mickey, Reference Nikitenko, Mickey, Beaudoin and Head2004; Zakharov et al. Reference Zakharov, Shurygin, Il’ina and Nikitenko2006).
The early Toarcian Age witnessed two large migrations of foraminifers and ostracods to Arctic and western European basins (Nikitenko & Mickey, Reference Nikitenko, Mickey, Beaudoin and Head2004; Nikitenko, Reference Nikitenko2008, Reference Nikitenko2009; Nikitenko et al. Reference Nikitenko, Shurygin and Mickey2008). The first migration occurred in the earliest Falciferum Chron associated with eustatic sea-level rise and considerable climate warming. Thermophylic microfauna that migrated to the Arctic Sea were not common and did not evolve there. In contrast, Arctic forms that migrated to the low-latitude seas often became successful in marginal environments. A second period of considerable migrations occurred in the Commune Chron (= Bifrons Chron; Fig. 6). Representatives of Arctic microfauna that migrated to western European seas often did establish themselves there. For example, representatives of Camptocythere (Ostracoda) appeared at the beginning of the early Toarcian Age (earliest Falciferum Chron) in the Arctic, migrated into western European seas through the Viking Corridor in the first half of the Commune Chron, and further existed there during the late Toarcian – Middle Jurassic interval (Triebel, Reference Triebel1950; Bate & Coleman, Reference Bate and Coleman1975; Ainsworth, Reference Ainsworth1986; Nikitenko & Mickey, Reference Nikitenko, Mickey, Beaudoin and Head2004; Nikitenko, Reference Nikitenko2009).
The northwards spread of low-latitude organisms has been interpreted to reflect the generally northwards flow through the Viking Corridor during much of the Early Jurassic Period (Korte et al. Reference Korte, Hesselbo, Ullmann, Dietl, Ruhl, Schweigert and Thibault2015). However, this is not reflected in geochemical proxy records and coupled ocean–climate models. Nd-isotopes obtained from Early Jurassic fish remains were used to determine the influence of the Viking and Hispanic corridors during the onset of the T-OAE, but diagenetic problems leading to a low-resolution dataset made it difficult to draw very firm conclusions (Dera et al. Reference Dera, Puceat, Pellenard, Neige, Delsate, Joachimski, Reisberg and Martinez2009b). However, Dera et al. (Reference Dera, Puceat, Pellenard, Neige, Delsate, Joachimski, Reisberg and Martinez2009b) did envision a predominantly southwards flow through the Viking Corridor during the Pliensbachian–Toarcian ages with relatively unradiogenic Arctic water entering the Tethys. Oxygen isotope records from belemnite calcite show a pronounced shift towards more negative δ18O values at the onset of the T-OAE, which has been attributed to a concomitant decrease in salinity and a temperature rise of up to 7°C (Bailey et al. Reference Bailey, Rosenthal, McArthur, van de Schootbrugge and Thirlwall2003). Belemnite oxygen isotope values remain relatively stable after the T-OAE, which Korte et al. (Reference Korte, Hesselbo, Ullmann, Dietl, Ruhl, Schweigert and Thibault2015) interpreted as reflecting stable temperatures in NW Europe. However, these authors ignore the influence of salinity changes on belemnite oxygen isotope values. We suggest that the apparent stability in oxygen isotope values could be driven by a change in salinity, whereby the influx of low-salinity waters masked temperature effects.
Reconstructions of circulation that rely on numerical models (Bjerrum & Surlyk, Reference Bjerrum and Surlyk2001; Dera & Donnadieu, Reference Dera and Donnadieu2012; Ruvalcaba Baroni et al. Reference Ruvalcaba Baroni, Pohl, van Helmond, Papadomanolaki, Coe, Cohen, van de Schootbrugge, Donnadieu and Slomp2018) have reached remarkably consistent conclusions about the direction of flow through the Viking Corridor. All these models show predominant southwards flow of low-salinity waters from the Arctic into the Tethys. The most up-to-date model presented by Ruvalcaba Baroni et al. (Reference Ruvalcaba Baroni, Pohl, van Helmond, Papadomanolaki, Coe, Cohen, van de Schootbrugge, Donnadieu and Slomp2018) indicates that, under high atmospheric pCO2 conditions such as during the Toarcian Age, oceanographic conditions in the EES were dominated by strong clockwise circulation in the Tethys bringing warm saline waters onto European shelves (Fig. 11). This strong circulation may have diminished the effects of flow through the Viking Corridor, which was further shunted by sedimentation, eustacy and tectonics in the corridor. Lowered pCO2 following the T-OAE may therefore have led to a decrease in the strength of Tethyan flow and enhanced the influence of Arctic influx. Such a change in dominant flow can be compared with a switch from an anti-estuarine circulation with inflowing warm saline waters from the Tethys, capped by low-salinity waters from run-off during the T-OAE, to an estuarine circulation with the influx of colder, nutrient- and oxygen-rich waters from the Arctic during the Bifrons Zone.
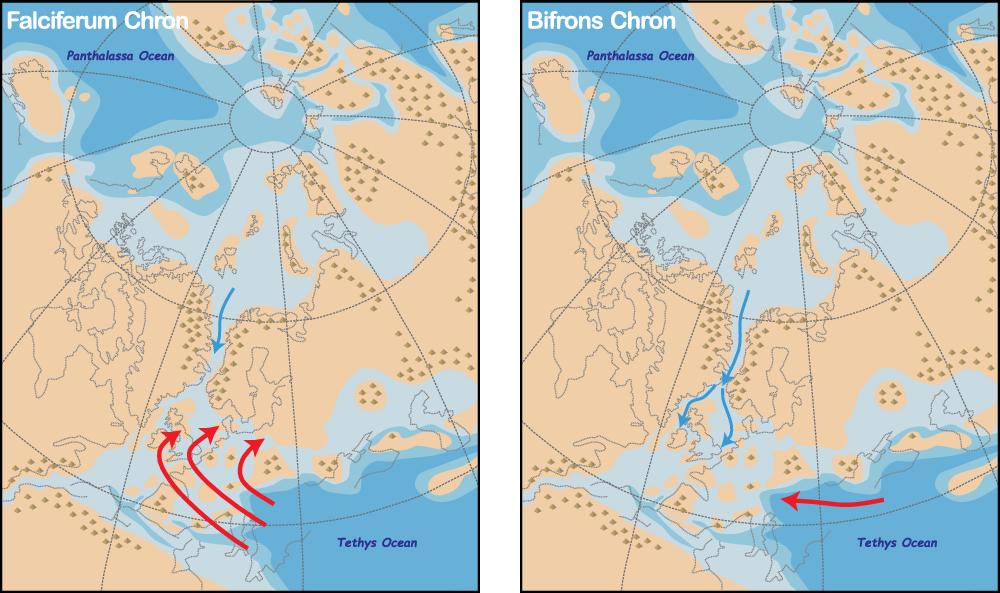
Fig. 11. Circulation through the Viking Corridor during the Falciferum and Bifrons chrons. During the Falciferum Chron, strong clockwise circulation in the Tethys brought warm saline waters onto the shelves that were capped by low-salinity waters from enhanced run-off. During the Bifrons Chron, reduced intensity of circulation in the Tethys and flooding of the Viking Corridor during a general highstand triggered the influx of colder low-salinity water from the Arctic, leading to the rapid spread of the Parvocysta–Phallocysta suite in NW Europe.
5.d. The end of an euxinic event in NW Europe
The transition from the early Toarcian Falciferum to Bifrons Chron was a major turning point in the development of the T-OAE in both the Arctic and the Tethyan realms and is clearly marked in biotic and geochemical proxy records. TOC values decrease across this boundary to more modest levels from their absolute highest values within the Exaratum Zone. Carbon isotope records show a positive excursion at this level that is generally masked in organic carbon isotope records by organic matter composition, but clearly shows up in many carbonate carbon isotope records (Suan et al. Reference Suan, van de Schootbrugge, Adatte, Fiebig and Oschmann2015). The enrichment of redox-sensitive elements has been widely used to reconstruct oxygenation and restriction of basins across the T-OAE (McArthur et al. Reference McArthur, Algeo, van de Schootbrugge, Li and Howarth2008). Mo and As show enrichment during the Falciferum Zone in Yorkshire, but values show a strong decline across the Falciferum–Bifrons transition (Thibault et al. Reference Thibault, Ruhl, Ullmann, Korte, Kemp, Gröcke and Hesselbo2018). Total sulphur and degree of pyritization show similar trends (Thibault et al. Reference Thibault, Ruhl, Ullmann, Korte, Kemp, Gröcke and Hesselbo2018).
Carbonate-associated sulphur isotope (δ34Scas) records obtained from belemnite calcite show a positive excursion within the upper part of the Falciferum Zone that has been correlated with the positive excursion in carbon isotope records (Gill et al. Reference Gill, Lyons and Jenkyns2011). Rather than declining to background values as organic matter burial and presumably associated pyrite burial dwindled, the δ34Scas isotope values remain generally very high from this point onwards in the UK and Germany for the duration of the Toarcian Age (Gill et al. Reference Gill, Lyons and Jenkyns2011; Newton et al. Reference Newton, Reeves, Kafousia, Wignall, Bottrell and Sha2011). The high values in δ34Scas for the upper Toarcian succession are puzzling because they are not matched by elevated organic carbon burial rates. Both Gill et al. (Reference Gill, Lyons and Jenkyns2011) and Newton et al. (Reference Newton, Reeves, Kafousia, Wignall, Bottrell and Sha2011) interpret these data to reflect the presence of low-salinity marine water with low sulphate concentrations. High-latitude water would be a major source of such water, and influx of this water through the Viking Corridor, could perhaps explain this anomalous signal. Interestingly, δ34Scas values return to more normal marine values during the Aalenian Age, presumably as the throughflow in the Viking Corridor slowed down or ceased.
Different biotic proxy records show an overall amelioration of oxygen conditions at the sea floor at the Falciferum–Bifrons transition. In Germany this boundary coincides with the deposition of a marker bed known as the Monotis Bed, named after abundant inoceramid bivalves at this level (van de Schootbrugge et al. Reference van de Schootbrugge, Richoz, Pross, Luppold, Hunze, Wonik, Blau, Meister, van der Weijst, Suan, Fraguas, Fiebig, Herrle, Guex, Little, Wignall, Püttmann and Oschmann2018). In southern Germany, prolonged colonization of the seafloor by benthic bivalves started at this level (Röhl et al. Reference Röhl, Schmid-Röhl, Oschmann, Frimmel and Schwark2001), and bioturbation increased in intensity and complexity at this level in the Cleveland Basin (Thibault et al. Reference Thibault, Ruhl, Ullmann, Korte, Kemp, Gröcke and Hesselbo2018). Ammonites show a high similarity between Arctic and Tethyan faunas during the Falciferum Zone, suggesting faunal exchange proceeded northwards, driven by global warming. During the Bifrons Zone ammonite faunas became markedly differentiated between high and low latitudes (Dera et al. Reference Dera, Neige, Dommergues and Brayard2011), perhaps indicating isolation of Arctic faunas as a result of predominant southwards flow through the Viking Corridor in addition to increasing latitudinal temperature gradients.
The transition from the Falciferum to the Bifrons Zone marked an end to the most intense anoxic conditions characterized by photic zone euxinia in Germany and the UK. In many southern European Tethyan locations, for example in Italy, Spain and Greece, organic matter accumulation was restricted to this interval in the lower part of the Falciferum (= Serpentinum Zone) and oxygenated conditions were re-established during the remainder of the Toarcian Age. However, in NW-European epeiric sea, deposition of organic-rich sediments continued throughout the Toarcian Age (van de Schootbrugge et al. Reference van de Schootbrugge, Richoz, Pross, Luppold, Hunze, Wonik, Blau, Meister, van der Weijst, Suan, Fraguas, Fiebig, Herrle, Guex, Little, Wignall, Püttmann and Oschmann2018). The prolongation of anoxic conditions in NW Europe for much of the Toarcian Age was likely directly linked to the arrival of low-salinity Arctic waters.
6. Conclusions
The combined use of carbon isotope records and dinoflagellate cyst assemblages from sites within and substantially north and south of the Viking Corridor allow us to shed light on the evolution of cyst-producing dinoflagellates during early Toarcian time, as well as their migration through the Viking Corridor. The Parvocysta–Phallocysta suite of dinoflagellates did not suddenly originate in the Boreal realm during the late Toarcian Age as has been suggested. Instead of being a rapid speciation event, palynological records from Siberia indicate that this important group of characteristic Early Jurassic cyst-forming dinoflagellates originated in the high latitudes in relatively cold Arctic waters, and were able to disperse southwards due to deepening of the Viking Corridor at the end of the Toarcian carbon cycle perturbation at the Falciferum–Bifrons Zone transition (Fig. 12). Arctic origins have also been surmised for other groups of dinoflagellates during the Triassic Period, and could be connected to dynamic nutrient and light conditions in the Arctic. Although the Siberian sections show evidence of black shale deposition, it is likely that conditions were more dynamic here than in lower-latitude sites with frequent mixing and re-oxygenation of the water column in relation to stark seasonal contrasts. This likely explains the high abundance and diversity of dinoflagellate cysts in the Arctic, even at the height of the Toarcian carbon cycle perturbation, much in contrast to NW Europe where a so-called dinoflagellate cyst black-out has been recognized during this interval. The spread of high-latitude phytoplankton following the T-OAE has implications for palaeoceanographic reconstructions for this time interval. Our data reveal that stratification during the T-OAE was not driven by Arctic influx, but rather by increased run-off. Instead, the switch from euxinic conditions to more dysoxic or even oxic conditions in NW Europe and the western Tethys could be explained by the arrival of cooler low-salinity nutrient-rich waters through the Viking Corridor, marking the end of the T-OAE.

Fig. 12. Range charts for selected genera showing southwards migration of the Parvocysta–Phallocysta suite into NW Europe and the Tethys. Phallocysta is absent from southern Europe. Early gonyaulacoid cysts occur within the Arctic and Viking Corridor, but appear only much later in NW Europe. The sudden appearance of the Parvocysta–Phallocysta suite coincides with the end of the most intense euxinic conditions across basins in the European Epicontinental Seaway.
Acknowledgments
We acknowledge support from Jonah Chietoli (NHM), Baerbel Schmincke (GUF) and Natasja Welters (UU) for their help with the processing of samples of the Kelimyar River. Results from Well 34/10-35 and the Cleveland Basin composite section were generated as part of the HYPO-Lias Project. This project was carried out by TNO and funded by Equinor ASA, Nederlandse Aardolie Maatschappij NAM, B.V. and Energie Beheer Nederland (EBN) B.V. This project also received a subsidy from the Ministry of Economic Affairs, National Regulations EA-subsidies, Topsector Energy executed by the Netherlands Enterprise Agency. Boris Nikitenko was supported by RSF 18-17-00038 and RFBR 18-05-70035 grants. BN also acknowledges support from project FNI 0331-2019-0005 and IGCP-655 (IUGS-UNESCO). We also thank Jim Riding and an anonymous reviewer for their helpful comments.