The majority of the Antarctic land surface is covered by ice and snow, resulting in only partial and scattered exposures of Cambrian carbonate platform deposits. Allochthonous archaeocyath-bearing clasts have, therefore, been crucial to establish both a regional biostratigraphic framework and to inform the palaeogeography between localities in Antarctica and southern Gondwana from the Cambrian through to the Late Palaeozoic glaciation records (Fig. 1). Nevertheless, at times, interpretations have been based solely on the presence of archaeocyaths. Meanwhile, the microfacies and diagenetic evolution of the Antarctic Cambrian carbonate platforms have been poorly studied.

Figure 1 Paleogeography of southern Gondwana during mid-Cambrian–late Carboniferous (modified from Boger Reference Boger2011) with the distribution of the archaeocyathan assemblages. Star outlined in red: record analysed in this study. E Antarctica region and W Antarctica terranes in dark grey colour. Abbreviations: EAC = East Antarctic Craton; SR = Shackleton Range; PM = Pensacola Mountains; WN = Whichaway Nunataks; cTAM = central Transantarctic Mountains; EWB = Ellsworth–Whitmore Block; AP = Antarctic Peninsula domains; MBL = Marie Byrd Land; TI = Thurston Island; Ar = Argentina; Nam = Namibia; Sa = South Africa; Fk = Falkland Islands; Sb = Stansbury Basin; Gs = Gnalta Shelf; Ab = Arrowie Basin.
Often in Antarctica, the finding of allochthonous archaeocyaths has preceded the location of the Cambrian carbonate successions they came from (Hill Reference Hill1965; Cooper & Shergold Reference Cooper, Shergold and Tingey1991 and references therein). In fact, archaeocyaths were the first Cambrian fossils collected in Antarctica by the Bruce's Scottish National Antarctic Expedition (1902–1904) from deep sea gravels at the Weddel Sea (station 313; see Pirie Reference Pirie1913) and by the Shackleton's British Antarctic Expedition (1907–1909) from moraine deposits of the Beardmore Glacier at the Transantarctic Mountains (TAM) (David & Priestley Reference David and Priestley1914). However, thick successions of Cambrian carbonate platforms only crop out along the TAM and in the Ellsworth Mountains (EW) at the W Antarctica region.
The intraplate TAM belt separates the E from W Antarctica regions (Fig. 2a) that present clear differences in their geological evolution. The E Antarctic Craton (EAC) is formed by the amalgamation of Precambrian terranes during different Precambrian–Cambrian orogenies (e.g., Boger Reference Boger2011). The geologic record of the TAM belt (see Goodge Reference Goodge2020) includes a Mesoarchaean and Paleoproterozoic basement (part of the EAC). This basement was rifted and developed an Andean-style Gondwana convergent margin with carbonate deposition (PM and cTAM in Fig. 1), basin inversion and clastic molasse-type sedimentation during the late Neoproterozoic to early Palaeozoic Ross orogenic cycle. Intra-cratonic and foreland basin sedimentation and mantle upwelling (Gondwana breakup) continued during Late Palaeozoic and Early Mesozoic times, and experimented rift-shoulder uplift related to Cenozoic extension (Goodge Reference Goodge2020). On the other hand, the W Antarctica extensional province is formed by Palaeozoic–Mesozoic microcontinental blocks (outboard terranes) as the Ellsworth–Whitmore Block (EWB), Thurston Island, Marie Byrd Land and the Antarctic Peninsula (Dalziel & Elliot Reference Dalziel and Elliot1982; EWB, TI, MBL, AP in Fig. 1) and both the Weddell Sea and W Antarctic rift systems (Fig. 2a). From ~500 Ma, the W Antarctica region is a tectonically active margin between the subducting paleo-Pacific oceanic plate and the EAC (Jordan et al. Reference Jordan, Riley and Siddoway2020 and references therein) (Fig. 1). Thus, the best known Cambrian carbonate successions in Antarctica (TAM and EW) were not part of the same carbonate platform, nor they did share a common tectonosedimentary evolution during the Cambrian – something that is key when establishing the origin of allochthonous archaeocyath-bearing clasts in the neighbouring regions of southern Gondwana (Fig. 1)

Figure 2 (A) Location of the Shackleton Range on the Antarctica continent. The E Antarctic plate is outlined by a thick black line to distinguish it from the W Antarctic region, which is formed by the amalgamation of several terranes. Abbreviations: AP = Antarctic Peninsula; EWB = Ellsworth–Whitmore Block; TI = Thurston Island; MBL = Marie Byrd Land; TAM = Transantarctic Mountains; CL = Coats Land; DML = Dronning Maud Land. (B) Simplified geologic map of the Shackleton Range. The inferred geology under the permanent snow and ice cover is based on the geology of scattered rock outcrops (1–8). Modified from Krohne et al. (Reference Krohne, Lisker, Kleinschmidt, Klügel, Läufers, Estrada and Spiegel2016) after Tessensohn et al. (Reference Tessensohn, Kleinschmidt, Talarico, Buggisch, Brommer, Henjes-Kunst, Kroner, Millar and Zeh1999a) and Kleinschmidt et al. (Reference Kleinschmidt, Buggisch, Läufer, Helferich and Tessensohn2002). Rock outcrop localities with archaeocyaths are indicated with numbers in bold: 1 = Otter Highlands; 2 = Haskard Highlands, 3 = La Grange Nunataks; 4 = Herbert Mountains; 5 = Pioneers Escarpment; 6 = Read Mountains; 7 = Du Toit Nunataks; 8 = Stephenson Bastion. Abbreviations: OHT = Otter Highlands Thrust; MWT = Mount Wegener Thrust. (C) Schematic n–S cross-section through the centre of the Shackleton Range. Tectonic units I–IV according to Tessensohn et al. (Reference Tessensohn, Kleinschmidt, Talarico, Buggisch, Brommer, Henjes-Kunst, Kroner, Millar and Zeh1999a), who interpreted the Shackleton collisional orogen as a result of the final amalgamation between E and W Gondwana during Late Precambrian–Cambrian time. I = Proterozoic basement; II = Ophiolitic complex; III = low-grade metasedimentary units with Cambrian fossils (including archaeocyaths) and Ross deformation ages; IV = Proterozoic basement (East Antarctic Craton) and autochthonous sedimentary cover.
On the other hand, the record of Antarctic allochthonous archaeocyathan assemblages is abundant, scattered and, at times, very far from the hypothetical source areas, and some of them have not yet been studied. However, because less than 2 % of the bedrock is exposed in Antarctica, archaeocyath-bearing clasts are key records that give us a chance to solve many geological problems. For instance, in the TAM, the presence of archaeocyath-bearing clasts in Cambrian conglomerates (e.g., Starshot Formation, Douglas Conglomerate) was useful to establish maximum depositional ages, lithostratigraphic relationships and relative timing of deformation, uplift and erosion of the Cambrian Series 2 carbonate platform (Rowell et al. Reference Rowell, Rees, Cooper and Pratt1986, Reference Rowell, Rees, Cooper and Pratt1988; Myrow et al. Reference Myrow, Pope, Goodge, Fischer and Palmer2002a). In recent tills, the presence of archaeocyath-bearing clasts derived from Cambrian polymictic conglomerates would indicate that the Douglas Conglomerate could be hidden under the ice at least as far S as the Beardmore Glacier (Rowell & Rees Reference Rowell and Rees1989).
In the EW (EWB in Fig. 1), the Heritage Group contains thick carbonate successions such as the middle Cambrian Drake Icefall Formation and the middle-upper Cambrian Minaret Formation (Webers et al. Reference Webers, Craddock and Splettstoesser1992). However, the only record of lower Cambrian carbonate sedimentation occurs as reworked carbonate clasts in the lower Cambrian conglomerates of the Kosco Peak Member (Castillo et al. Reference Castillo, Fanning, Fernández, Poblete and Hervé2017) from the Heritage Group and in Permo-Carboniferous tillites of the Whiteout Conglomerate (Buggisch & Webers Reference Buggisch and Webers1992). In fact, the Whiteout Conglomerate represents the Late Palaeozoic Gondwana glaciation sedimentation in W Antarctica (Matsch & Ojakangas Reference Matsch, Ojakangas, Webers, Craddock and Splettstoesser1992). But the source area of the carbonate clasts is unknown in the EW; specifically, the archaeocyath-bearing clasts give a lower Cambrian age (Debrenne Reference Debrenne1992) and no clasts believed to be from the Minaret Formation are found (Buggisch & Webers Reference Buggisch and Webers1992).
In Late Palaeozoic Gondwana glaciation deposits outside Antarctica, the presence of archaeocyath-bearing clasts (Fig. 1) allows paleobiogeographic correlations between Antarctica and other erratic assemblages in S Gondwana where the record of Cambrian carbonate successions is unknown, as in the Falkland Islands (Stone et al. Reference Stone, Thomson and Rushton2012), South Africa (Debrenne Reference Debrenne1975), Namibia (Perejón et al. Reference Perejón, Rodríguez-Martínez, Moreno-Eiris, Menéndez and Reitner2019) and Argentina (González et al. Reference González, Tortello, Damborenea, Naipauer, Sato and Varela2013). In this regard, the autochthonous archaeocyathan assemblages from the lower Cambrian Shackleton Limestone (TAM; Hill Reference Hill1964b; Debrenne & Kruse Reference Debrenne and Kruse1986, Reference Debrenne and Kruse1989), the Schneider Hills limestone (Pensacola Mountains (PM), TAM; Konyushkov & Shulyatin Reference Konyushkov, Shulyatin and Zhuravleva1980; Debrenne & Kruse Reference Debrenne and Kruse1989), the middle Cambrian Nelson Limestone (PM; Wood et al. Reference Wood, Evans and Zhuravlev1992) or the upper Cambrian Minaret Formation (EWB; Debrenne et al. Reference Debrenne, Rozanov and Webers1984; Henderson et al. Reference Henderson, Debrenne, Rowell, Webers, Webers, Craddock and Splettstoesser1992) are, so far, essential to establish the likely source areas of allochthonous records. But, are these the only Cambrian carbonate successions, or are there other records hidden under the ice? And, if so, how can we infer and differentiate them?
The Shackleton Range (SR in Fig. 1) is interpreted as a collisional orogen due to the final amalgamation between E and W Gondwana during Late Precambrian–Cambrian times (Tessensohn et al. Reference Tessensohn, Kleinschmidt, Talarico, Buggisch, Brommer, Henjes-Kunst, Kroner, Millar and Zeh1999a), but the presence of shallow-water marine successions from the Cambrian is unknown in this sector. However, glacial erratics with Cambrian brachiopods from a locally sourced area were the first fossils collected by the Shackleton Range geological expedition in recent moraine deposits (1970–1971; Clarkson Reference Clarkson1971; Thomson Reference Thomson1972). And later, during the German geological expedition GEISHA (1987–1988), Cenozoic glacial erratic archaeocyath-bearing clasts were discovered. Höfle & Buggisch (Reference Höfle and Buggisch1995) suggested that a major expansion of the Antarctic Ice Sheet carried these erratic carbonate clasts from their hypothetical source area: the Nelson Limestone in the PM, the southernmost part of the TAM (PM in Fig. 1). Some years later, during the EUROSHACK expedition (1993–1994), a sampling of new archaeocyath-bearing clasts from the Mount Wegener Formation was carried out. Buggisch & Henjes-Kunst (Reference Buggisch and Henjes-Kunst1999) suggested an early Cambrian Atdabanian age for the synorogenic upper slope to basinal deposits of the Mount Wegener Formation on the basis of the potassium–argon (K–Ar) ages of detrital muscovites and the presence of the trace fossil Oldhamia, calcimicrobes and archaeocyaths. Therefore, the carbonate clasts from the Mount Wegener Formation come from the erosion of shallow marine deposits; although geochemical and paleocurrent data suggest a northern source area, its final provenance is not yet determined (Buggisch & Henjes-Kunst Reference Buggisch and Henjes-Kunst1999).
The aims of this paper are: (a) to analyse the microfacies of the Cambrian carbonate clasts from the Shackleton Range (Cenozoic tills and Cambrian Mount Wegener Formation); (b) to identify the diagenetic processes that carbonate clasts and host rock conglomerates have undergone, from the shallow marine platform stage up to the very low-grade metamorphic overprint and nappe-tectonic stage; (c) to analyse the taxonomic relationships of the archaeocyath specimens; (d) to determine the age of archaeocyathan assemblage to set the minimum age of the carbonate platform they derived from; (e) to establish possible palaeobiogeographic correlations between the archaeocyathan assemblage from the Shackleton Range sector and other coeval assemblages; (f) to give new age constraints of the Mount Wegener Formation; (g) to figure out the different depositional sub-environments of the lost carbonate platform in the Shackleton Range sector; and (f) to compare this lost carbonate platform and its tectonosedimentary evolution with other contemporary Antarctic platforms.
1. Geological setting
The Shackleton Range (lat. 80°–81°S, long. 19°–31°W) is the most prominent massif at the Coats Land region of Antarctica, extending for about 200 km in an E–W direction. The range is bounded by the Slessor and Recovery glaciers that drain the E Antarctic Ice Sheet into the Filchner Ice Shelf (Weddell Sea) (Fig. 2a, b). Above permanent snow and ice, the closest outcrops/blocks are the Whichaway Nunataks to the S (130 km), the Argentina Range at the PM to the SW (240 km) and the Theron Mountains to the N (150 km). The first geological survey of the western part of the Shackleton Range was carried out by Stephenson (Reference Stephenson1966) during the Trans-Antarctic Expedition (1955–1958). Later, the geology of the range was successively studied by numerous field parties from the UK, USSR, Germany, Italy and the USA (see Clarkson Reference Clarkson, Thomson and Thomson1995; Kleinschmidt Reference Kleinschmidt and Riffenburgh2007; Fig. 3 and references therein).
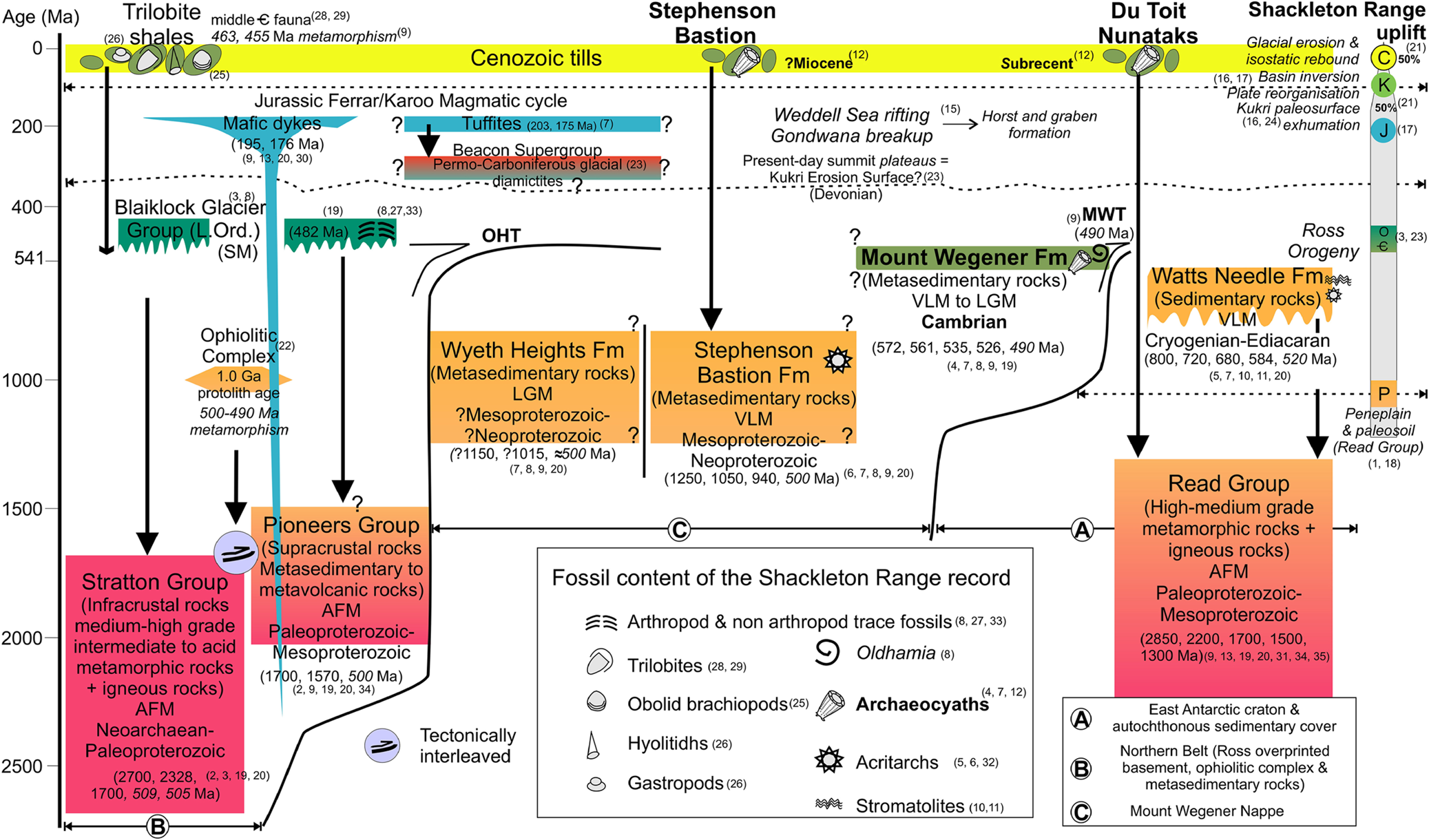
Figure 3 Chronostratigraphic chart of the Shackleton Range showing the main lithostratigraphic units and their fossil content. The Shackleton Range can be subdivided in three tectonic units (Ⓐ, Ⓑ, Ⓒ). The major uplift phases of the Shackleton Range are summarised on the right. Abbreviations: OHT = Otter Highlands Thrust; MWT = Mount Wegener Thrust; AFM = Amphibolite facies metamorphism; LGM = low-grade metamorphism; VLGM = very low-grade metamorphism; SM = sedimentary molasse deposits; ? = relationships between lithostratigraphic units are not always observed due to permanent snow and ice cover and hence are uncertain. The Cambrian record corresponds to the Mount Wegener Formation and the Cenozoic tills. The analysed Cambrian carbonate clasts belong to the Mount Wegener Formation and to the Cenozoic tills from the Stephenson Bastion and Du Toit Nunataks. Ages, lithology, fossil content and uplift data are based on the following publications: 1 = Clarkson (Reference Clarkson1982); 2 = Brommer et al. (Reference Brommer, Millar and Zeh1999); 3 = Buggisch et al. (Reference Buggisch, Bachtadse and Henjes-Kunst1999); 4 = Buggisch & Henjes-Kunst (Reference Buggisch and Henjes-Kunst1999); 5 = Buggisch et al. (Reference Buggisch, Höhndorf, Kreuzer, Paech, Weber, Thomson and Thomson1995a); 6 = Buggisch et al. (Reference Buggisch, Höhndorf, Paech, Kleinschmidt, Kreuzer, Weber, Thomson and Thomson1995b); 7 = Buggisch et al. (Reference Buggisch, Kleinschmidt, Höhndorf and Pohl1994a); 8 = Buggisch et al. (Reference Buggisch, Kleinschmidt, Kreuzer and Krumm1990); 9 = Buggisch et al. (Reference Buggisch, Kleinschmidt, Kreuzer and Krumm1994b); 10 = Golovanov et al. (Reference Golovanov, Milstein, Mikhailov and Shulyatin1979); 11 = Golovanov et al. (Reference Golovanov, Mikhailov and Shulyatin1980); 12 = Höfle & Buggisch (Reference Höfle and Buggisch1995); 13 = Hofmann et al. (Reference Hofmann, Kaiser, Klemm and Paech1980); 14 = Hotten (Reference Hotten1993); 15 = Jordan et al. (Reference Jordan, Ferraccioli and Leat2017); 16 = Krohne et al. (Reference Krohne, Lisker, Kleinschmidt, Klügel, Läufers, Estrada and Spiegel2016); 17 = Lisker et al. (Reference Lisker, Schäfer and Olesch1999); 18 = Paech (Reference Paech and Lange1982); 19 = Pankhurst et al. (Reference Pankhurst, Marsh, Clarkson, Oliver, James and Jago1983); 20 = Pankhurst et al. (Reference Pankhurst, Kreuzer, Höhndorf, Belyatsky, Thomson and Thomson1985); 21 = Paxman et al. (Reference Paxman, Jamieson, Ferraccioli, Bentley, Forsberg, Ross, Watts, Corr and Jordan2017); 22 = Talarico et al. (Reference Talarico, Kleinschmidt and Henjes-Kunst1999); 23 = Tessensohn (Reference Tessensohn and Ricci1997); 24 = Tessensohn et al. (Reference Tessensohn, Kleinschmidt and Buggisch1999b); 25 = Thomson (Reference Thomson1972); 26 = Thomson et al. (Reference Thomson, Solov'ev, Buggisch, Thomson and Thomson1995); 27 = Thomson & Weber (Reference Thomson and Weber1999); 28 = Solov'ev & Grikurov (Reference Solov'ev and Grikurov1979); 29 = Cooper & Shergold (Reference Cooper, Shergold and Tingey1991); 30 = Spaeth et al. (Reference Spaeth, Hotten, Peters and Techmer1995); 31 = Rex (Reference Rex and Adie1972); 32 = Weber (Reference Weber1991); 33 = Weber & Brady (Reference Weber and Brady2004); 34 = Zeh et al. (Reference Zeh, Millar and Horstwood2004); 35 = Will et al. (Reference Will, Zeh, Gerdes, Frimmel, Millar and Schmädicke2009).
The geology of the range consists mainly of medium–high-grade amphibolite facies of the Proterozoic Shackleton Range Metamorphic Complex (SRMC) (Clarkson Reference Clarkson1972), which is made of infracrustral rocks from the Neoarchaean–Paleoproterozoic Stratton Group (Tessensohn & Thomson Reference Tessensohn and Thomson1990) and the Paleoproterozoic–Mesoproterozoic Read Group (Pankhurst et al. Reference Pankhurst, Marsh, Clarkson, Oliver, James and Jago1983, Reference Pankhurst, Kreuzer, Höhndorf, Belyatsky, Thomson and Thomson1985; Fig. 3). The snow and ice cover made it difficult to establish lithostratigraphic relationships between the scattered rock outcrops; in fact, only two sedimentary contacts have been observed between the Proterozoic SRMC and the younger sedimentary successions, disregarding the Cenozoic deposits (Fig. 3). However, the nappe- and thrust-tectonic soon became apparent and finally well established (Marsh Reference Marsh, Oliver, James and Jago1983; Buggisch et al. Reference Buggisch, Kleinschmidt, Kreuzer and Krumm1990; Brommer Reference Brommer1998; Kleinschmidt et al. Reference Kleinschmidt, Henjes-Kunst and Tessensohn2001). Therefore, the Shackleton Range is subdivided into different tectonostratigraphic units developed during the Ross orogeny (Buggisch et al. Reference Buggisch, Kleinschmidt, Kreuzer and Krumm1994b; Buggisch & Kleinschmidt Reference Buggisch and Kleinschmidt1999; Tessensohn et al. Reference Tessensohn, Kleinschmidt, Talarico, Buggisch, Brommer, Henjes-Kunst, Kroner, Millar and Zeh1999a; Fig. 2c, A–C in Fig. 3).
The metasedimentary to metavolcanic supracrustal rocks of the Pioneers Group (Tessensohn & Thomson Reference Tessensohn and Thomson1990; Roland et al. Reference Roland, Braun, Hofman, Kamenev, Kamenenva, Kleischmidt, Olesch, Paech, Thomson and Thomson1995) and the rocks of the Ophiolitic Complex (Talarico et al. Reference Talarico, Kleinschmidt and Henjes-Kunst1999) with eclogite facies metamorphism (Schmädicke & Will Reference Schmädicke and Will2006) occur in the northern part of the range. Both appear tectonically interleaved with the Stratton Group (Fig. 3; Schubert et al. Reference Schubert, Braun, Kamenec, Olesch, Thomson and Thomson1995), forming the Northern Belt (B in Fig. 3). In the southern part of the range, the Read Group (Tessensohn & Thomson Reference Tessensohn and Thomson1990; Olesch et al. Reference Olesch, Braun, Kamenev, Kameneva, Schubert, Thomson and Thomson1995), which is part of the EAC, is unconformably overlaid by the Watts Needle Formation (Marsh Reference Marsh, Oliver, James and Jago1983), interpreted as Criogenian–Ediacaran marine mixed platform sedimentation (A in Fig. 3). The boundaries between the northern and southern belts are thrusts (OHT and MWT in Figs 2b, 3), which bound the Mount Wegener Nappe (Kleinschmidt & Buggisch Reference Kleinschmidt and Buggisch1994; Buggisch & Kleinschmidt Reference Buggisch and Kleinschmidt2007 and references therein). This allochthonous tectonic unit comprises very-low- to low-grade metasedimentary rocks (Clarkson Reference Clarkson1972; C in Fig. 3) as the ?Mesoproterozoic to Neoproterozoic Wyeth Heights and Stephenson Bastion Formations, and the Cambrian Mount Wegener Formation, which hosts allochthonous archaeocyath-bearing clasts in conglomerates and olistoliths (Buggisch et al. Reference Buggisch, Bachtadse and Henjes-Kunst1999; Buggisch & Henjes-Kunst Reference Buggisch and Henjes-Kunst1999). The Shackleton Range is a collisional orogen related to the sinistral collision between the E Antarctic and Kalahari Cratons with the closure of the Mozambique Ocean during the final amalgamation of the E and W Gondwana (Talarico et al. Reference Talarico, Kleinschmidt and Henjes-Kunst1999; Tessensohn et al. Reference Tessensohn, Kleinschmidt, Talarico, Buggisch, Brommer, Henjes-Kunst, Kroner, Millar and Zeh1999a; Kleinschmidt et al. Reference Kleinschmidt, Henjes-Kunst and Tessensohn2001). This late Pan-African event produced the westward overthrusting of the Northern Belt and the southward overthrusting of the Mount Wegener Nappe over their foreland, the EAC (Read Group) and its sedimentary cover (Watts Needle Formation) according to Buggisch & Kleinschmidt (Reference Buggisch and Kleinschmidt2007).
The subsequent Blaiklock Glacier Group (Clarkson Reference Clarkson1972; Clarkson & Wyeth Reference Clarkson and Wyeth1983) consists of post-orogenic Ordovician red beds and molasses (Figs 2b, 3 and references therein), interpreted as alluvial, fluvial to coastal deposits (Buggisch et al. Reference Buggisch, Bachtadse and Henjes-Kunst1999). This group unconformably overlies a Pan-African overprinted basement (Stratton Group) in the northwestern and western part of the Northern Belt. In the easternmost area of the range (Fig. 2b), a small outcrop of non-fossiliferous Permian tillites, considered the basal part of the Beacon Supergroup (Tessensohn et al. Reference Tessensohn, Kleinschmidt and Buggisch1999b), are unconformably overlaid by Upper Triassic–Lower Jurassic volcano-sedimentary deposits (Buggisch et al. Reference Buggisch, Kleinschmidt, Kreuzer and Krumm1994b; Krohne et al. Reference Krohne, Lisker, Kleinschmidt, Klügel, Läufers, Estrada and Spiegel2016). These volcano-sedimentary rocks have similar geochemistry and/or ages than those observed in dolerite/mafic dykes of the nearby La Grange Nunataks (3 in Fig. 2B), the Whichaway Nunataks and the Theron Mountains. Thus, they are considered part of the Ferrar/Karoo magmatic cycle (Buggisch et al. Reference Buggisch, Kleinschmidt, Höhndorf and Pohl1994a; Krohne et al. Reference Krohne, Lisker, Kleinschmidt, Klügel, Läufers, Estrada and Spiegel2016). The geochemistry of the N–S strike Jurassic dolerite dykes from La Grange Nunataks corresponds to the initial rifting tholeiites linked with the mafic rocks of the Ferrar Group during the break-up of the Gondwana supercontinent (Spaeth et al. Reference Spaeth, Hotten, Peters and Techmer1995). Thus, the younger record of the Shackleton Range is formed by Jurassic dikes and tuffites and Cenozoic tills (Figs 2b, 3 and references therein). The occurrence of Cenozoic tills with Cambrian fauna-bearing clasts has been described in three different locations. At the first locality, the Haskard Highlands (2 in Fig. 2b), shale boulders in moraine contain middle Cambrian fauna (Trilobite shales in Fig. 3). The second and third localities are in the southern part of the range, where the archaeocyaths occur as part of Cenozoic tills at the Du Toit Nunataks and the Stephenson Bastion (7 and 8 in Figs 2b, 3).
2. Stratigraphic record of the allochthonous archaeocyaths in the Shackleton Range
In the Shackleton Range, the ice divide separates tributary glaciers that flow northwards to the Slessor Glacier from those that flow southwards to the Recovery Glacier. The archaeocyath-bearing clasts are found at the S of the Fuchs Dome (central ice cap) and the Shotton Snowfield as glacial erratics in Cenozoic tills and conglomerates hosted by the Cambrian Mount Wegener Formation (Fig. 4a, b).

Figure 4 (A) Location of glacial erratics and in situ archaeocyath-bearing clasts in the Shackleton Range. The main rock outcrops (1–8), some tributary glaciers (S1–3, R1–2) and the current direction of ice flows are shown (modified from Höfle & Buggisch Reference Höfle and Buggisch1995). Box outlines area of (B). Abbreviations: 1 = Otter Highlands; 2 = Haskard Highlands; 3 = La Grange Nunataks; 4 = Herbert Mountains; 5 = Pioneers Escarpment; 6 = Read Mountains; 7 = Du Toit Nunataks; 8 = Stephenson Bastion; S1 = Blaiklock Glacier; S2 = Straton Glacier; S3 = Gordon Glacier; R1 = Cornwall Glacier; R2 = Glen Glacier. (B) Partial geological map of the southern Shackleton Range (modified from Clarkson et al. Reference Clarkson, Tessensohn, Thomson, Belyatsky, Braun, Buggisch, Grikurov, Hofmann, Höhndorf, Kamenev, Kameneva, Kleinschmidt, Kreuzer, Leat, Olesch, Paech, Pankhurst, Peters, Roland, Schubert, Solov'ev, Spaeth, Techmer, Thomson, Weber, Weber and King1995). Black stars: in situ archaeocyath-bearing clasts are found in Cambrian marine slope deposits of the Mount Wegener Formation (Buggisch et al. Reference Buggisch, Kleinschmidt, Höhndorf and Pohl1994a; Buggisch & Henjes-Kunst Reference Buggisch and Henjes-Kunst1999). Green stars: glacial erratic archaeocyath-bearing clasts occur as part of pre-Quaternary till deposits (Stephenson Bastion) and recent frontal moraines (Du Toit Nunataks). Boxes outline areas of (C) and (D). Abbreviations: OHT = Otter Highlands Thrust; MWT = Mount Wegener Thrust. (C) Detail from (B) showing the location of glacial erratic archaeocyath-bearing clasts according to Höfle & Buggisch (Reference Höfle and Buggisch1995). The late Precambrian Stephenson Bastion Formation emerges in a plateau landform. Archaeocyaths occur as part of pre-Quaternary till deposits on the plateau. The pre-Quaternary till deposits on the Stephenson Bastion plateau, erosive structures and forms (striated rock surfaces, roches moutonnées, etc.) suggest that the overriding ice flowed north-westward (see the text). The overriding ice directions are shown according to Kerr & Hermichen (Reference Kerr and Hermichen1999) and Sugden et al. (Reference Sugden, Fogwill, Hein, Stuart, Kerr and Kubik2014). (D) Detail from (B) showing the location of the sampling sites with archaeocyaths within the Mount Wegener Formation. The exact location of the samples with archaeocyaths from Oldhamia Terraces is unknown. Key: 1 = conglomerates; 2 = greywackes; 3 = shales; 4 = olistoliths; 5 = Oldhamia ichnotaxon. Geological map and sedimentary palaeocurrents of the Mount Wegener Formation according to Buggisch & Henjes-Kunst (Reference Buggisch and Henjes-Kunst1999). The black bar represents the stratigraphic section (see Fig. 5). Abbreviations for (A) and (C): MP = Mount Provender; WH = Wyeth Heights; MG = Mount Greenfield; MW = Mount Wegener.
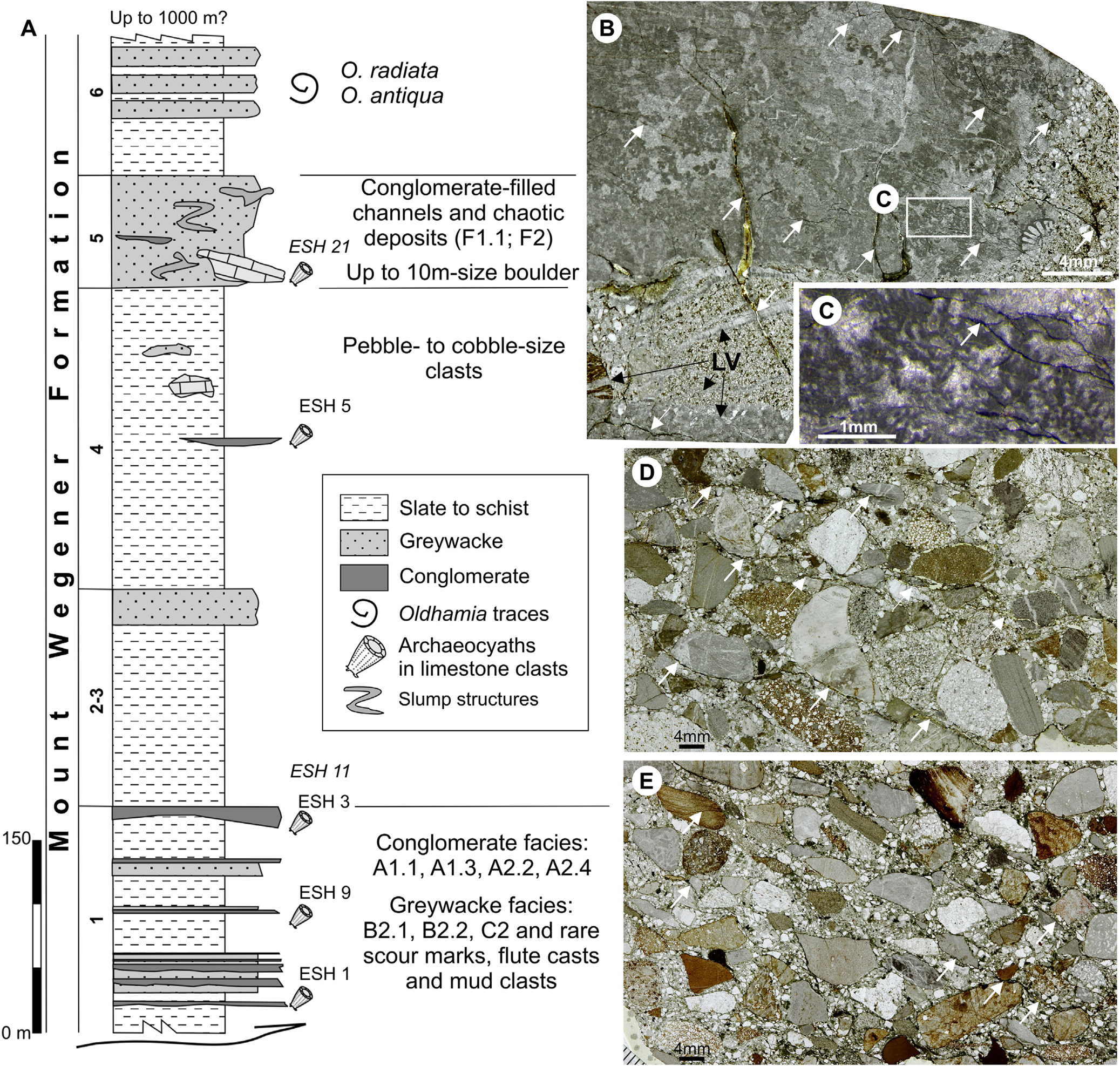
Figure 5 (A) Stratigraphic section of the lower part of the Mount Wegener Formation in Trueman Terraces (total thickness probably exceeds 1000 m) and distribution of facies codes (modified from Buggisch & Henjes-Kunst Reference Buggisch and Henjes-Kunst1999). Facies codes according to Pickering et al. (Reference Pickering, Stow, Watson and Hiscott1986): A1.1 = stratified gravels; B2.1 = parallel-stratified sands; B2.2 = cross-stratified sands; C2 = organised sand-mud couplets; F1.1 = rubble; F2 = contorted/disturbed strata. Only the stratigraphic position of the samples with archaeocyaths (ESH Trueman Terraces) and those with probable lateral equivalence (ESH Swinnerton Ledge) is shown. (B–E) Thin-section photomicrographs (plane-polarised light) of polymictic conglomerates of the Cambrian Mount Wegener Formation in Oldhamia Terraces (B) and Trueman Terraces (D) and conglomerate clast of the Cenozoic tills on the Stephenson Bastion (E) (see Fig. 4). (B) Calcimicrobe-rich limestone pebble floating in a sandy matrix. Clasts and sandy matrix are cut by a late vein fracture system (LV) that is cut by an incipient rough cleavage fabric (white arrows). (C) Detail Epiphyton and cements crossed by a late cleavage fabric (white arrow). (D–E) Note the similarities in clast composition and tectonic deformation (white arrows: cleavage, irregular sutured grain boundaries) in both conglomerates. In these examples, conglomerate clast from the Cenozoic tills (E) show more continuous cleavage traces around strongly tectonically oriented grains.
The glacial erratic archaeocyath-bearing clasts (Fig. 4a–c) were discovered in the Shackleton Range during the German geological expedition GEISHA (1987–1988). At that time, the presence of archaeocyath-bearing clasts in the conglomerates of the Cambrian Mount Wegener Formation was unknown (Fig. 4b, d) and knowledge about the uplift of the Shackleton Range and the glaciology of the region was limited. Höfle & Buggisch (Reference Höfle and Buggisch1995) analysed the glacial morphology and till deposits from the Shackleton Range. Some of the data and conclusions are summarised below. The glacial erratic archaeocyath-bearing clasts occur in two different situations (Fig. 3): as Cenozoic tills on top of the Stephenson Bastion table mountain (Fig. 4c) and as part of a subrecent moraine near Du Toit Nunataks (Fig. 4b). The archaeocyath-bearing clasts consist of wackestone, floatstone and boundstone with Epiphyton, Renalcis, Batenevia ramosa Korde and trilobites. Many of the outcrops at the Shackleton Range are table mountains, relics of an exhumed peneplain (Stephenson Reference Stephenson1966; Skidmore & Clarkson Reference Skidmore and Clarkson1972; Marsh Reference Marsh1985). The scarce till deposits on the table mountains occur in places protected from erosion. In fact, easily weathered fossiliferous limestone clasts appear in hollows and depressions. On the relict till deposits on top of the Stephenson Bastion table mountain (Fig. 4c), the archaeocyath-bearing clasts are subsidiary clasts while the reddish-brown ?Beacon Supergroupsandstone derived clasts and local bed rock fragments (greenish-grey quartzite derived from the Stephenson Bastion Formation) are dominant. Subglacial erosional forms on the Stephenson Bastion table mountain (Fig. 4c) suggest that overriding ice covered the western part of Shackleton Range and flowed NW (Höfle & Buggisch Reference Höfle and Buggisch1995; Kerr & Hermichen Reference Kerr and Hermichen1999; Sugden et al. Reference Sugden, Fogwill, Hein, Stuart, Kerr and Kubik2014). Höfle & Buggisch (Reference Höfle and Buggisch1995) hypothesised that the Cambrian exotic erratics on the Stephenson Bastion were generated during a major expansion of the Antarctic Ice Sheet at the end of Miocene. They suggested the Whichaway Nunataks as source area for the exotic ?Beacon Supergroup erratics. For Cambrian archaeocyath-bearing clasts, they suggested the Whichaway Nunataks and the PM, which have early to middle Cambrian limestones. Kerr & Hermichen (Reference Kerr and Hermichen1999) interpreted the southeastern flows as an expansion of the Recovery Glacier and those from the SW as an expansion of the Filchner-Ronne Ice Shelf. They concluded that there is no evidence for extensive glacial modification of the Shackleton Range plateau by the E Antarctic Ice Sheet or by Quaternary ice. Cosmogenic nuclide data indicate that the glacial overriding of the higher summits of the Shackleton Range and deepening of the Slessor and Recovery glaciers troughs was earlier than 2.5 Ma, and significant erosion occurred in the mid-Miocene maximum (Sugden et al. Reference Sugden, Fogwill, Hein, Stuart, Kerr and Kubik2014).
The archaeocyath-bearing clasts occur in conglomerates and boulders of the Mount Wegener Formation, which is part of the Mount Wegener Nappe. Buggisch & Henjes-Kunst (Reference Buggisch and Henjes-Kunst1999) reported archaeocyaths from E of the Read Mountain at the Oldhamia, Trueman Terraces and the Swinnerton Ledge (Fig. 4d). They described bioclastic rudstone and grainstone, archaeocyath/calcimicrobe boundstone microfacies with diverse biota such as archaeocyaths, trilobites, mollusc, echinoderms and calcimicrobes (Epyphyton, Korilophyton, Renalcis granosus Vologdin, Renalcis seriata Korde, Girvanella, Batenevia and Subtifloria) and concluded that the carbonate clasts represent the breakup and destruction of shallow-water carbonate deposits.
3. Mount Wegener Formation
Broadly, the Mount Wegener Formation comprises shales/slates, greywackes and conglomerates, and shows folding and metamorphic overprint that increases from SE to NW (Buggisch et al. Reference Buggisch, Kleinschmidt, Kreuzer and Krumm1994b). The total thickness of the Mount Wegener Formation probably exceeds 1000 m, and stratigraphy (see Fig. 5), facies, geochemistry and provenance analysis was carried out by Buggisch & Henjes-Kunst (Reference Buggisch and Henjes-Kunst1999) with data obtained during the EUROSHACK expedition (1993–1994). The main results and conclusions are summarised below. The facies of the Mount Wegener Formation are interpreted as marine clastic deposits from upper slope to deep basin set up in synorogenic conditions, the presence of plagioclase and volcaniclastics support an intracontinental back-arc environment (Buggisch et al. Reference Buggisch, Kleinschmidt, Höhndorf and Pohl1994a). The clast composition of the conglomerates shows evidence of repeated erosion and redeposition in shallow-water conditions before their final sedimentation as marine slope deposits. Reworked sedimentary rocks are dominant, while basement-derived rocks are rare. The most abundant clasts are sandstone, carbonate and conglomerate. The sandstones (greywackes) from the Mount Wegener Formation present intermediate proportions of silicon dioxide/aluminium oxide and low potassium oxide/sodium oxide ratios like those observed in the passive continental margins. Indeed, isotope geochemistry (ɛNd,530Ma values) suggests that the supracrustal rocks of the Pioneers Group (the Northern Belt, a Ross/Pan-African overprinted basement; Fig. 3) could be a potential source of sediments from the Mount Wegener Formation. The K–Ar dating ages of detrital muscovites between 572 and 534 Ma also suggest basement rocks with Pan-African cooling histories as the main source.
The age of the Mount Wegener Formation was interpreted by Buggisch et al. (Reference Buggisch, Kleinschmidt, Kreuzer and Krumm1994b) as early Cambrian based on the presence of fossils (Oldhamia cf. antiqua and Oldhamia cf. radiata, Epiphyton sp. and ?Botomaella sp.) and on the rubidium–strontium (Rb–Sr) analysis (561 ± 18 Ma and 539 ± 9 Ma isochrones; Fig. 3 and references therein). The structural data and K–Ar analysis on phyllosilicates (2–6 μm whole rock fraction) indicate that the low-grade metamorphic overprint and the southwards transport of the Mount Wegener Nappe occurred around 490 Ma as a result of the Ross (Pan-African) orogeny (Buggisch et al. Reference Buggisch, Kleinschmidt, Kreuzer and Krumm1994b).
4. Materials and methods
The carbonate clasts and archaeocyath-bearing limestone clasts samples described here were collected during the GEISHA (First German geological expedition to the Shackleton Range) and EUROSHACK (European Antarctic Geological Expedition) expeditions. These geoscientific land expeditions took place in the Shackleton Range during 1987/1988 and 1994/1995, respectively. GEISHA was jointly supported by the Alfred Wegener Institute for Polar and Marine Research (AWI) and Federal Institute for Geosciences and Natural Resources (BGR), whereas EUROSHACK was under the leadership of the BGR and British Antarctic Survey (BAS).
In the GEISHA expedition, about 300 samples were collected to assess the degree of metamorphism, and approximately 200 thin sections were studied with this purpose (Buggisch et al. Reference Buggisch, Kleinschmidt, Kreuzer and Krumm1994b). In the EUROSHACK expedition, about 150 samples were taken from 43 different stratigraphic levels and/or localities from the Mount Wegener Formation. They were used to analyse facies, provenance, geochemistry and K–Ar dating. Most of these samples come from conglomerates or limestone clasts within the conglomerates (Buggisch & Henjes-Kunst Reference Buggisch and Henjes-Kunst1999).
A total of 237 petrographic thin sections have been reviewed in this study (GEISHA and EUROSHACK samples): 45 from the Stephenson Bastion and Du Toit Nunataks and 188 from the Read Mountains (Fig. 4b). GEISHA thin sections are from the Stephenson Bastion (9) and Du Toit Nunataks (2). EUROSHACK thin sections are from the Stephenson Bastion (34) and the Mount Wegener Formation (189) in the eastern part of the Read Mountains: Oldhamia (91) and Trueman (50) terraces, Swinnerton Ledge (47). In addition, four thin sections of indeterminate origin from EUROSHACK have been reviewed.
Out of 237 petrographic thin sections, 223 were medium in size (5 × 5 cm2) and 14 were small (2.7 × 4.8 cm2). The microfacies and palaeontological analyses were performed with petrographic and binocular microscopes. The samples were classified according to the schemes of Dunham (Reference Dunham1962), Embry & Klovan (Reference Embry and Klovan1971) and Wright (Reference Wright1992). The peloid subcategories are according Flügel (Reference Flügel2004) and the sediment grain sizes according Wentworth (Reference Wentworth1922) size classes. The percentage of allochems was semi-quantitatively analysed by visual estimation charts (several authors in Flügel Reference Flügel2004). The basic types of porosity and pore-size classes were described following the classification of Choquette & Pray (Reference Choquette and Pray1970). Selected thin sections were stained with alizarin red sulphur and potassium ferricyanide to analyse their staining response (calcite/dolomite and iron content, respectively) according to Dickson (Reference Dickson1965, Reference Dickson1966). The classification of dolomite crystal fabrics follows the Sibley & Gregg (Reference Sibley and Gregg1987) scheme and the crystal-size scale for carbonate rocks is according to Folk (Reference Folk1962). A total of 367 archaeocyath specimens and one coralomorph have been recognised from 46 thin sections. Among archaeocyathan specimens, 189 were classified following the systematics of the Treatise on invertebrate paleontology (Debrenne et al. Reference Debrenne, Zhuravlev, Kruse and Selden2015).
The studied material is housed in the Museo Geominero (MGM), Instituto Geológico y Minero de España (IGME, Spanish Geological Survey, Madrid) under the code numbers MGM-7202X–MGM-7438X.
5. Carbonate clasts record from the Cambrian Mount Wegener Formation and the Cenozoic tills
The analysed carbonate clasts from the Cambrian Mount Wegener Formation and the Cenozoic tills (Stephenson Bastion and Du Toit Nunataks) show equivalent components and microfacies. The analysed carbonate clasts are accessory to primary components in the sandy polymictic conglomerates and breccias from the lower Cambrian Mount Wegener Formation and from the Cenozoic tills. The carbonate clasts correspond to limestones and dolostones (see sections 5.1 and 5.2) where the main allochems are non-skeletal grains, skeletal grains and calcimicrobes. The non-skeletal grains include aggregate grains, mud peloids (micritic intraclasts), bahamite peloids (round micritic grains), algal peloids (calcimicrobe-derived micritic grains) and different types of ooids (types 1–3). Very fine to fine-grained size superficial ooids with quartz nuclei are type 1 ooids. Medium-sized and very coarse to granule-sized ooids are type 2 and type 3, respectively. The carbonate clasts from the Mount Wegener Formation and Cenozoic tills also show equivalent early to late diagenetic phases and tectonic-induced fabrics (Fig. 5). The main diagenetic processes recorded in carbonate clasts and their host rocks (conglomerates) are described in section 6.
In addition to the similarities observed in the microfacies, diagenetic and tectonic processes, the size distribution of the archaeocyathan cups has been analysed to assess whether or not the allochthonous archaeocyaths from the Cenozoic tills (Stephenson Bastion and Du Toit Nunataks) are derived or not from the Mount Wegener Formation (Fig. 6). The diameter of the archaeocyathan cups is measured in transverse section from the thin sections. A total number of 189 specimens have been analysed (see section 7) and 181 specimens have been measured from the Stephenson Bastion/Du Toit Nunataks (70) and the Mount Wegener Formation (111). Some archaeocyaths cups are distorted by tectonic stresses (see section 6). In these cases, the minimum diameter has been selected to reduce bias due to tectonically strained archaeocyath cups and characterise the size distribution of archaeocyaths. The archaeocyathan fauna from the Cenozoic tills shows the same average diameter as those observed in the Mount Wegener Formation (3.25 mm), as well as equivalent median values (2.65 and 2.85 mm, respectively; see Fig. 6a, b). Therefore, the analysis of cup sizes supports a single source for the archaeocyaths from the Cenozoic tills and the Mount Wegener Formation. In addition, we have grouped all the measured archaeocyaths into a single set to analyse how the cup sizes are distributed. We have selected three diameter size ranges: small (0.2–2.9 mm), medium (3.0–5.9 mm) and large (6.0–14 mm). In the Shackleton Range, more than half of the specimens are small in size (53 %), and the rest are mainly medium in size (34 %), while the largest sizes are minority (13 %) (Fig. 6c). Most archaeocyaths have a size diameter ranging from 10 to 50 mm (Rowland Reference Rowland2001). Furthermore, typical conical cups of Ajacicyathidae are around 5–15 mm (Debrenne et al. Reference Debrenne, Zhuravlev and Kruse2012). Cordie & Dornbos (Reference Cordie and Dornbos2019) have measured the traditional morphometric characters of more than 1000 archaeocyaths, mainly from the orders Ajacicyathida and Archaeocyathida. They have reported an average diameter of 10.6 mm and a median value of 8.67 mm (0.78 mm minimum to 74 mm maximum). Cordie & Dornbos (Reference Cordie and Dornbos2019) reported an average diameter of 10.6 mm from more than 1000 measured archaeocyaths. Therefore, the archaeocyaths from the Shackleton Range record are small compared to published data. This trend in a smaller sized fauna for Antarctic record has also been observed in Cambrian helcionelloids from the Shackleton Limestone (central TAM) that were compared with helcionelloid mollusc assemblages from the Ajax Limestone (Australia) and the Bastion Formation (Greenland) (Jackson & Claybourn Reference Jackson and Claybourn2018).

Figure 6 Distribution of the archaeocyath diameters from the Shackleton Range. (A) Diameter sizes graph of the archaeocyathan cups from Cenozoic tills located at the Stephenson Bastion and Du Toit Nunataks. (B) Diameter sizes graph of the archaeocyathan cups from the Mount Wegener Formation (Oldhamia and Trueman Terraces and Swinnerton Ledge). (C) Pie chart showing the three stablished ranges of measurements of archaeocyathan cup diameters.
All these data from the analysis of microfacies, diagenetic processes and archaeocyathan diameter sizes confirm that Cenozoic carbonate erratics from the Stephenson Bastion and Du Toit Nunataks are derived from the Cambrian Mount Wegener Formation. Thus, the analysis of carbonate clasts from the Shackleton Range provides crucial information about the history of a hidden Cambrian carbonate platform from which they were derived.
5.1. Dolostone clasts microfacies (DM)
The analysed dolostone clasts (Figs 7, 8a) have been arranged in three main categories according to the percentage of quartz grains, the proportion of non-skeletal grains or the predominance of a dolomite crystal fabric as: (DMa) dolomitic sandstones to sandy dolostones (two and four subcategories, respectively); (DMb) aggregate-grain- to ooid-rich dolostones (three subcategories); and (DMc) dolostones.
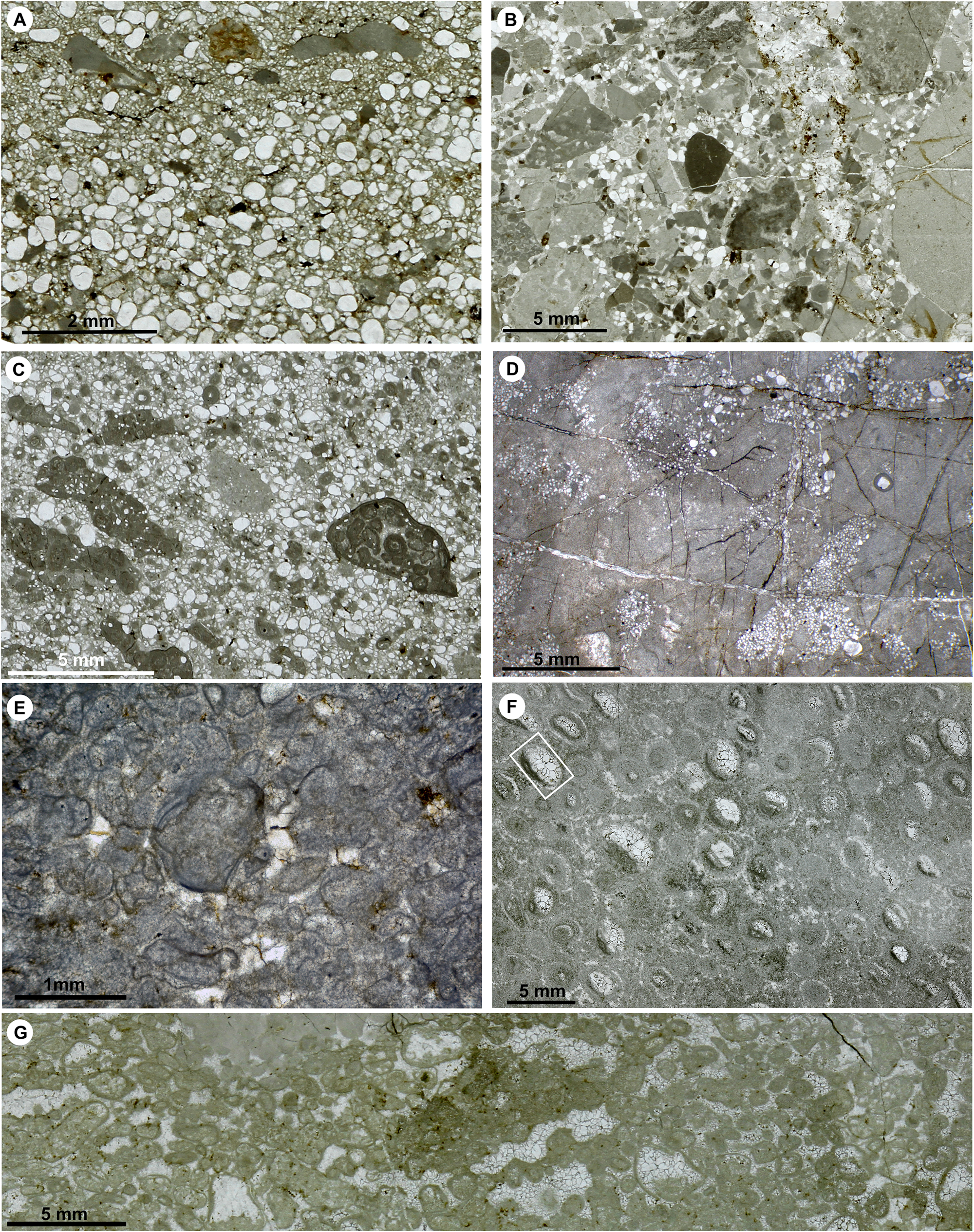
Figure 7 Cambrian dolostone clast microfacies from the Cambrian Mount Wegener Formation and the Cenozoic glacial erratics. Dolomitic sandstone: (A) coarse, poorly sorted, intraclastic, dolomitic sandstone. (B–D) Sandy dolostones (B) coarse, very poorly sorted, massive, clast-supported, intraclastic dolorudite; (C) medium, poorly to moderately sorted, oolitic, intraclastic, dolorudite; (D) dolomicrite with large megapores with siliciclastic filling. (E–G) Aggregate grain- to ooid-rich dolostones (E) aggregate grain dolopackstone; (F) loosely packed oolitic dolopackstone (the inset corresponds to Fig. 9b); (G) fenestral, aggregate grain-oolitic dolograinstone.

Figure 8 Cambrian dolostone and limestone clasts microfacies from the Cambrian Mount Wegener Formation and the Cenozoic glacial erratics. (A) Dolostone with meso- and megapores filled with multiple phases of dolomite cements, including black bitumen. The boxes correspond to Fig. 10a–c. Calcimicrobes from calcimicrobial-rich microfacies: (B) Angusticellularia dendrolitic microframe; (C) Epiphyton dendrolitic microframe; (D) broken Renalcis bunches surrounded by detrital grains; (E) Botomaella fan-like forms; (F, G) Epiphyton–Girvanella intergrowth. (H, I) Tarthinia–Epiphyton–Girvanella intergrowth. (J) Calcimicrobe grainstone clast with Subtifloria remains.

Figure 9 Diagenetic processes recorded in the Cambrian carbonate clasts of the Cambrian Mount Wegener Formation and the Cenozoic tills. (A–E) Dolostone clasts showing different processes of dolomitisation and silicification. (A) Detail of oolitic dolograinstone showing evidence of early mimetic dolomitisation of ooids (RD1), early marine fibrous and equant phreatic cements (M1RD1 and M2RD1, respectively). (B) Detail of oolitic dolopackstone (see Fig. 7f) where the oomoldic porosity is filled with an early authigenic silica phase (Schc = chalcedony cement) and a fracture-related silica phase (Sqtz = megaquartz mosaic). (C) The replacive cryptocrystalline to microcrystalline silicification (RS1c-m) post-dates early marine phreatic cements and mimetic dolomitisation and predates D2 dolomitisation stage (RD2). Megaquartz mosaics (Sqtz) are associated with late cement veins (LVA and LVB). (D) LVA (quartz–calcite vein) system with non-ferroan to slightly ferroan poikilotopic calcite cement (PkCc). (E) LVA system post-dates the dolomitisation stage D4. (F) Scheme with some of the main diagenetic processes recorded in the dolostone clasts. (G–L) Limestone clasts. (G) Detail of the archaeocyath cementstone with large fabric selective porosity infilled by early marine phreatic cement (M1Cc = fibrous calcite cement; M2Cc = bladed to equant non-ferroan calcite cement) and late diagenetic phases (D4). (H) Archaeocyathan intervallum (left) showing irregular vugs that post-date early marine phreatic cements. (I) Epiphyton microframe with secondary non-fabric selective porosity with geopetal sedimentary fillings (SI). Note how the growth polarity of the calcimicrobe (red arrowhead) does not match the polarity of the geopetal fillings (black arrowhead). Note how the stylolites (white arrows) post-date the sedimentary infillings. (J) Polymictic conglomerate showing a large peloid-intraclastic-bioclastic grainstone clast with enlarged fenestrae. Megapores are filled with sedimentary fillings (SI1 and SI2) and (K) drusy mosaic with compositional zoning starting with non-ferroan calcite and ending with fracture-related ferroan calcite cement. White box outlines area of (L). (L) Sedimentary filling (SI2) is composed of sandy-silty matrix (identical to the host rock conglomerate matrix) and eroded crystals derived from the non-ferroan calcite cement.

Figure 10 Late diagenetic and tectonically induced fabrics in Cambrian carbonate clasts of the Mount Wegener Formation and the Cenozoic tills. Details of dolostone with large vuggy to channelised cavities with multi-episodic fillings, locations of (A)–(C) in Fig. 8a. (A) Burial dolomites D2 and D3. D3 is rich in black bitumen residues. (B) D3 dolomite crust grading into epitaxial D4 stage. Late cement vein (LVA) with quartz (Sqtz) and poikilotopic non-ferroan to slightly ferroan calcite (PkCc) cuts the pore-lining dolomite crust cements (D2, D3, D4). (C) Late cement vein (LVA) cuts the remains of black bitumen from the cavity, thus post-dating the burial D2–D4 dolomites and the migration of hydrocarbons. (D) Detail of late cement vein (LVB) with ferroan saddle dolomite (FeD), megaquartz crystals (Sqtz) and zoned ferroan calcite cements (FeCc). (E) Late cement vein (LVB). Note the euhedral terminations of the megaquartz crystals and the posterior superimposed tectonically induced cataclastic fabric (white arrows). (F) Detail of late cement vein (LVB) that crosses the sandy matrix of the polymictic conglomerate. Notice how the remaining intergranular porosity of the matrix is filled with ferroan calcite cement. Note the posterior superimposed tectonically induced cataclastic fabric (black arrows). (G) Detail of the zoned ferroan calcite cement showing type III calcite twins. (H) Calcimicrobe boundstone where secondary porosity has been filled with quartz (Sqtz) and poikilotopic non-ferroan to slightly ferroan calcite (PkCc), which are both late fracture-related cements (LVA). Notice how the second fracture system (LVB) is posterior to the LVA cements. (I) Sparstone clast where it is possible to recognise the inner and outer walls of an archaeocyathan cup. Note the presence of partial replacement processes such as dolomitisation (Dol) and silicification (Sqtz). (J) Archaeocyath cementstone where early marine cementation is crossed by late cement veins (LVB) with dolomite (Dol) and ferroan calcite (FeCc). White box outlines are of (K). (K) Detail of archaeocyathan intervallum. Note how dolomitisation is associated with hair-like fractures with ferroan carbonate fluids and is higher in substrates with precursor mineralogies with high magnesium content, such as archaeocyaths. (L, M) Tectonically induced fabrics in limestone clast (L) and conglomerate (M).
The presence of very well-rounded quartz sand grains is characteristic in the dolomitic sandstones to sandy dolostones. Dolomitic sandstones range from fine to medium very well-sorted dolomitic sandstone (DMa1) to coarse, poorly sorted intraclastic dolomitic sandstone (DMa2) (Fig. 7a). In the first one, quartz grains reach up to 65 % of the rock volume. In the second one, they represent 40–50 %, while subrounded dolomicrite and dolospar intraclasts reach 30 % of the rock volume.
The sandy dolostones are coarse intraclastic dolorudites (DMa3), medium oolitic-intraclastic dolorudites (DMa4) and dolomicrites (DMa5–6), and their quartz grain content is in the range 1–30 % of the rock volume. In the sandy, coarse, very poorly sorted massive clast-supported intraclastic dolorudites (DMa3) (Fig. 7b), subangular to well-rounded intraclasts (up to 1.7 cm) are polygenic. Dolomicrites and silty dolomicrites are dominant intraclasts (up to 60 % of the rock volume), while dolograinstones rich in ooids, aggregate-grains or algal peloids are minor intraclasts. Oolitic dolograinstone intraclasts are made up of different types of ooids (types 1–3). In addition, fragments of type 3 oolitic cortices, abraded micritic-coated clasts and orange fibrous cement crusts also occur. Dominant coarse, very well-rounded quartz (up to 1 mm) and minor fine to medium subangular quartz sand grains are up to 25 % of the rock volume. Calcimicrobe intraclasts with Proaulopora and ?Renalcis are accessories.
In the sandy, medium poorly to moderately sorted oolitic-intraclastic dolorudites (DMa4) (Fig. 7c), elongated intraclasts are imbricated. Sandy oolitic (type 2) compound granule-size intraclasts account for about 35 % of the rock volume, while silty dolomicrite intraclasts are around 15 %. Superficial ooids (type 1) are 15 % of the rock volume. Quartz content corresponds to coarse very well-rounded grains (10 %) and fine to medium subangular grains (20 %).
The most common sandy dolomicrites (DMa5) are massive, with very accessory dispersed silt to very fine quartz grains (1 %). However, there are others (DMa6) with rare scattered pelletoids, superficial ooids (5 %) and accessory silt-sized quartz grains (10–15 %) that show the characteristic non-fabric-selective porosity (20 % of the rock volume). These large megapores (>1 cm) configure a network of irregular vuggy to channelised cavities with clastic fillings (Fig. 7d). The sedimentary fillings are primarily angular to subangular silt-size quartz grains, very fine- to medium sand-sized superficial ooids and minor well-rounded medium sand-sized quartz grains.
Aggregate grain- to ooid-rich dolostones (DMb) are dominated by aggregate grains or ooids or a mixture of non-skeletal grains. Different cements and replacements of dolomite and silica occur in primary interparticle porosity and secondary selective leaching of the cortex and nuclei of non-skeletal grains, resulting in varying degrees of preservation of the original fabrics (see section 6). Compound grains, 200 μm to 1 mm in size, with grape-like to lobate shapes and irregular dark micritic envelopes, are forming aggregate grain dolowackestones to dolopackstones (DMb1) (Fig. 7e) with very accessory small superficial ooids (type 1). Laminar micritic concentric type 3 ooids (up to 5 mm, ‘giant ooids' sensu Sumner & Grotzinger Reference Sumner and Grotzinger1993 rather than pisoids) can form loosely packed oolitic dolowackestones to packed dolograinstones (DMb2) (Fig. 7f). These microfacies show moderately sorted to well-sorted simple ooids, with both spherical and ellipsoidal shapes. Symmetrical cortices predominate and broken cortices that act as nuclei are very common. Very well-rounded quartz grains (650 μm) are accessory in matrix and within compound ooids. Oolitic dolograinstones with very well-sorted silicified type 2 ooids (medium-grained sand size) also occur. The very poorly sorted aggregate grain-oolitic dolograinstone (DMb3) (Fig. 7g) shows a distinctive regular to irregular fenestral fabric (up to 1.5 cm wide) that is filled with geopetal infills and cements. In this microfacies, ooids are mixture of 1–2–3 types with different preservations. In the fenestral aggregate grain-oolitic dolograinstone are found large compound intraclasts, up to 5 mm wide, with aggregate grains and peloids as intracomponents and multiple micritic envelopes.
Dolostones with cemented non-fabric-selective porosity also occur (DMc). They are very fine to finely crystalline dolomites with intercrystalline mesopores and very large megapores (16 mm × 41 mm, vug to channel types). Large vuggy to channelised cavities can reach up 25–30 % of the rock volume and display multi-episodic fillings (Fig. 8a; see section 6).
5.2. Limestone clasts microfacies (LM)
The most frequent limestones are calcimicrobe- and calcimicrobe–archaeocyath-bearing clasts (63 thin-sections). Calcimicrobes and/or archaeocyaths are primary to accessory allochems that form calcimicrobial boundstone (LMd, 12.7 % of 63 thin-sections), calcimicrobial boundstone with archaeocyaths (LMe1, 5.5 %), calcimicrobe–archaeocyath boundstone (LMe2, 6.3 %), archaeocyath cementstone (LMe3, 15.9 %) and archaeocyath floatstone (LMf, 9.5 % of 63 thin-sections). Bioclastic wackestone to packstone (LMg) and peloid-intraclastic-bioclastic packstone to grainstone (LMh) are uncommon.
In the boundstone microfacies, calcimicrobes correspond to Angusticellularia (also named Angulocellularia), Epiphyton, Renalcis, Tarthinia, Girvanella and Botomaella (Fig. 8). Epiphyton or Angusticellularia are the main dendrolitic microframe-builders, while the archaeocyaths range from 10 to 25 %, 25 to 30 %, up to 40 to 50 % of the rock volume in the calcimicrobial boundstone with archaeocyaths, calcimicrobe–archaeocyath boundstone and archaeocyath cementstone, respectively. In the boundstone microfacies dominated by Epiphyton or Angusticellularia dendritic microframes (Fig. 8b, c), the associated skeletal components, mainly archaeocyaths or calcimicrobes, are extremely low. Few clusters of Renalcis (Fig. 8d) appear irregularly distributed among the dendrolites of Angusticellularia or Epiphyton or attached to the walls of archaeocyaths, which may be exceptionally covered by Girvanella crusts.
Epiphyton–Girvanella or Epiphyton–Tarthinia–Girvanella intergrowths form complex microframes, but they are not very common (Fig. 8f–i). Botomaella fan-shaped radiant filaments/tubes (Fig. 8e) are extremely rare in the boundstone microfacies. Tarthinia-dominated microframes are rare but are found surrounded by wackestone to packstone pockets with a rich associated fauna of echinoderms, brachiopods, trilobites, hyoliths, chancelloriids, coralomorphs and sponge megascleres. This rich associated fauna has also been observed in the calcimicrobe–archaeocyath boundstone microfacies. However, in the archaeocyath cementstone, the associated fauna is restricted to accessory hyoliths and chancelloriids.
The archaeocyath-bearing microfacies are clearly dominated by regular archaeocyaths (149 specimens versus 40 irregular specimens). In addition, in the archaeocyath cementstone the presence of irregular archaeocyaths is totally accessory. Regarding archaeocyaths distribution, a total number of 16 families of archaeocyaths occur in the boundstones (see Appendix 1). Rotundocyathus glacius, Cadniacyathus sp. and Archaeopharetra irregularis are the dominant species. Fifteen families are represented in the calcimicrobial boundstone with archaeocyaths, six in the calcimicrobe–archaeocyath boundstones and seven in the archaeocyath cementstones. Ajacicyathidae is the dominant family in the archaeocyath cementstones and calcimicrobial boundstones with archaeocyaths. The calcimicrobe–archaeocyath boundstones are dominated by Densocyathidae and Ajacicyathidae. Common families in all boundstones are Ajacicyathidae, Densocyathidae, Shackletoncyathidae, Loculicyathidae and Archaeopharetridae, while Kaltatocyathidae are only found in the calcimicrobe–archaeocyath boundstones.
The presence of skeletal components, other than archaeocyaths, in the rest of the analysed microfacies (limestone- or dolostone-derived clasts) is very rare in terms of volume and/or frequency. The archaeocyath floatstone consists of poorly sorted reworked archaeocyaths (25–35 % of the rock volume), hyoliths (1–5 % rock volume), allochthonous calcimicrobe remains (Proaulopora, Subtifloria) and calcimicrobial boundstone intraclasts (Renalcis- or Epiphyton-dominated microframes). The archaeocyath debris varies from septum to large cup remains. The archaeocyathan walls are mostly free of calcimicrobe encrustations but are unusually encrusted by Girvanella. Eleven families of archaeocyaths are represented in the archaeocyath floatstones – Bronchocyathidae and Ajacicyathidae are the dominant ones. Bronchocyathidae, Kymbecyathidae, Dictyocyathidae and Copleicyathidae are only found in the floatstones (see Appendix 1). Thalamocyathus trachealis is dominant in the floatstones.
In the peloid-intraclastic-bioclastic packstone to grainstone, fine- to medium-grained sand size, algal peloids, mud peloids and bioclasts are the most common allochems. The largest bioclasts correspond to archaeocyathan remains (>1 mm), while indeterminate thin-walled, conical crenulated shells and hyoliths are minor bioclastic components. This microfacies (Fig. 9j–l) contains small to enlarged fenestral megapores that are filled with characteristic sedimentary fillings and cements (see section 6).
6. Principal diagenetic processes and tectonic fabrics recorded in the carbonate clasts from the Cambrian Mount Wegener Formation and the Cenozoic tills
6.1. Early diagenetic phases
The dolomitic sandstones, sandy dolostones and aggregate grain- to ooid-rich dolostones display evidence of early marine phreatic cementation, such as yellow fibrous to bladed isopachous rims (M1RD1) and pore-filling equant cement (M2RD1) (Fig. 9a, f). Meteoric vadose diagenesis processes, such as partial dissolution producing secondary moldic porosity, are common in aggregate grain- to ooid-rich dolostones. Some samples show partial to complete intraparticle dissolution and internal collapse that produced geopetal infills in aggregate grains and ooids (‘half-moon’ ooids sensu Wherry Reference Wherry1916). The evidence of selective leaching (oomoldic porosity and selective destructive replacive fabrics of some cortical laminae; Figs 7f, 9c) suggests a differentiation of the primary mineralogical composition of the ooids (high magnesium calcite, aragonite). Likewise, fenestral aggregate grain-oolitic dolograinstones display dark microcrystalline crusts and meniscus fabrics with distinctive rounded pores (Fig. 7g) produced during meteoric vadose diagenesis (Dunham Reference Dunham and Bricker1971; Longman Reference Longman1980).
Many dolostone microfacies exhibit early pervasive mimetic dolomitisation of allochems (e.g., concentric ooids, aggregate grains), micrite and early marine phreatic and vadose cements (Figs 7, 9a–f). Early mimetic dolomitisation (RD1) predates compaction and is followed by different stages of dolomitisation and silicification linked with secondary porosity and fractures (Figs 9a–f; see section 3). In the aggregate grain- to ooid-rich dolostones an early authigenic and diagenetic phase of silica occurs as chalcedony void-filling cement (Schc) within the remaining interparticle and/or within the secondary moldic intraparticle porosities (e.g., oomoldic porosity, Fig. 9b). Chalcedony cement post-dates early mimetic dolomitisation (RD1) and predates all other fracture-related cements. Some cortices and nuclei from ooids and aggregate-grains exhibit replacive cryptocrystalline and microcrystalline silica fabrics (RS1c-m in Fig. 9c, d, f).
The calcimicrobial boundstone with archaeocyaths, calcimicrobe–archaeocyath boundstone and archaeocyath cementstone exhibit the largest fabric-selective porosity that is filled with early marine phreatic cementation. The inter-, intra-skeletal and growth framework meso- and megapores are filled with yellow, inclusion-rich, fibrous calcite cements (M1Cc) and bladed to equant non-ferroan calcite mosaics (M2Cc) that precede the formation of stylolites. In the archaeocyath cementstones, the isopachous marine fibrous calcite crusts (up to 2.5 mm thick) constitute around 30–40 % of the rock volume (Fig. 9g). Early marine phreatic cementation occluded a significant part of the primary mesopores in the calcimicrobe- and archaeocyath-bearing clasts. Nevertheless, the larger fabric-selective porosity registered successive diagenetic phases (see below).
6.2. Breakup and brecciation of platform and downslope transport of carbonate clasts
Irregular vugs with very angular silt to sand-sized crystal and quartz grain sedimentary fillings post-date early marine phreatic cements (M1Cc and M2Cc) in boundstone microfacies (Fig. 9h). Furthermore, the orientations of the geopetals within irregular vugs and the calcimicrobe microframework show inconsistent polarity relationships between them (Fig. 9i). Therefore, these irregular vugs cut and eroded the early pre-existing marine cements and the calcimicrobe microframework, suggesting meteoric diagenesis related to the breakup and sedimentary brecciation of the carbonate platform. These sedimentary fillings are equivalent to those observed in enlarged fenestral megapores developed in the peloid-intraclastic-bioclastic grainstone clasts (Fig. 9j).
The enlarged fenestral megapores are filled with two sedimentary fillings (SI1, SI2 in Fig. 9j) and drusy mosaic cement with crystals showing compositional zoning. Drusy zoned mosaic cement begins with non-ferroan calcite (pink-stained) and ends with ferroan calcite (mauve-stained) (Fig. 9k). However, not all megapores are occluded by the complete succession of drusy zoned mosaic cement. The sedimentary fills correspond to an early very fine-grained geopetal crystal silt (SI1) that is followed by a second fill (SI2). The latter fill has angular silt to sand-sized quartz grains and eroded crystals derived from the non-ferroan calcite drusy mosaic (Fig. 9l). The SI2 is observed in those fenestral pores close to the margins of the limestone clast. Furthermore, SI2 is equivalent to the surrounding conglomerate matrix (Fig. 9j). Thus, SI2 was infiltrated into the still-open fenestral pores during sedimentation of the carbonate clasts as part of the matrix-supported polymictic gravels on the marine slope, after the early non-ferroan calcite drusy mosaic. The succeeding ferroan calcite cement is fracture-related cement and occludes the remaining fenestral porosity (Fig. 9k).
6.3. Late diagenetic phases and mechanical overthrust-emplacement processes
In general, clasts in the sandy polymictic conglomerates from the Mount Wegener Formation and Cenozoic tills show dominant tangential contacts due to their deposition as matrix-supported gravels. However, the contacts between sandy matrix and clasts are sutured due to tectonic deformation (Fig. 5d, e). In carbonate clasts, mechanical compaction features were inhibited mostly by early cementation (M1, M2), dolomitisation (RD1) and silicification (Schc and RS1c-m) (Fig. 9). Thus, loosely packed fabrics are the most common, except for sandy intraclastic dolorudites (Fig. 7c), where tangential and long contacts dominate and there are also concavo–convex contacts between quartz and dolomite grains. Evidence of chemical compaction occurs as simple to sutured stylolites in some limestone clasts. These stylolites post-date both shallow marine cements (M1Cc, M2Cc) as well as geopetal sedimentary infillings produced during the downslope transport of the clasts.
Burial dolomites correspond to three different stages of dolomitisation (D2, D3 and D4). Stage D2 consists of coarsely crystalline (0.25–0.5 mm up to 1 mm), planar-s to planar-e type dolomite (types according to Sibley & Gregg, Reference Sibley and Gregg1987). D2 occurs mainly as replacive/cement dolomite in the interparticle, secondary intraparticle, intercrystal and fracture-associated porosities of the aggregate grain- to ooid-rich dolostones. D2 post-dates silica Schc cement and RS1c-m replacement (Fig. 9c, f). Stage D2 is also the first dolomite cement in the vug to channel cemented dolostones where the large megapores are lined by multiple isopachous layers of cement crusts (1.5–2.5 mm thick) (Figs 8a, 10a, b). In some cases, cavity-cement crusts may start with early yellow inclusion-rich fibrous cement (like those previously described as M1Cc) but now being a dolomite, so it could represent early mimetic dolomitisation of a fibrous marine aragonite/calcite cement precursor (M1RD1). However, most mesopores, megapores and fractures are rimmed by a first generation of cloudy to clear, coarsely crystalline (>0.25 mm) rhombic D2 dolomite (Fig. 10a). The boundary between the rhombic crystals D2 and the next stage D3 can be locally irregular and rich in black hydrocarbon residues. Stage D3 corresponds to a black inclusion-rich zoned, fibrous to elongated-bladed dolomite crust (up to 2 mm thick) (Fig. 10a). In some cavities, the D3 dolomite crusts show a clearer, less inclusion-rich epitaxial late D4 stage (Fig. 10b). There are traces of bitumen after the growth of D4 dolomite. Therefore, the hydrocarbon migration post-dates the D2 and D4 dolomite stages and was coeval with the D3 dolomite stage. In addition, the partial migration of the trapped hydrocarbon in the D3 cement left an open microporosity that was filled with the fracture-related cement. Stage D3 is not observed in the aggregate grain- to ooid-rich dolostones (Fig. 7e–g). However, a possible equivalent to stage D4 is recorded as a fracture-related phase of brown, thick twinning, very coarsely crystalline (1–4 mm), planar-s dolomite in the aggregate-grain to ooid-rich dolostones (Fig. 9e, f) and in the archaeocyath cementstones and floatstones (Fig. 9g). The abundance of black bitumen inclusions in D3–D4 suggests that they are burial diagenetic dolomites. The occurrence of black bitumen in mesopores and megapores from dolostones and the observed cross-cutting relationships suggest that hydrocarbon migration predates the void-late-fracture-related remnant cements (LVA, late cement vein system A) (Figs 8a, 10c). The appearance of black bitumen residues is recorded in thin hair-like fractures, intercrystalline mesopores and megapores in dolostones, calcimicrobial boundstones and polymictic conglomerates.
The late cement veins correspond to different mineral-filled fracture systems (LVA and LVB, respectively). The LVA system consists of quartz-calcite veins and the LVB system consist of (ferroan saddle dolomite)-quartz-ferroan calcite-quartz veins. LVA and LVB fluids precipitated in remnant open porosity in dolostone and limestone clasts and host rock conglomerates. Cross-cutting relationships, mineralogies and fabrics support LVB postdating LVA. In the LVA system (Fig. 9d, f), dissolved silica fluids first produced chert and microquartz replacement from the host rock and later precipitated as megaquartz mosaics within the remaining porosity and fractures. Second, dissolved non-ferroan to very slightly ferroan carbonate fluids precipitated as very to extremely coarse poikilotopic calcite cement with thin twin planes (and minor thick twin planes) in pores and fractures. In the LVB system, the fracture walls are sometimes lined with ferroan saddle dolomite relics (turquoise/greenish stain and curved faces; FeD in Fig. 10d) and, generally, by megaquartz crystals with euhedral terminations, which are embedded by coarsely to very coarsely crystalline zoned ferroan calcite with tabular thick twin planes (FeCc in Fig. 10d–g). Dissolved silica fluids from the LVB system also produced partial and selective host rock cryptocristalline and microcrystalline silica replacement (RS2c-m), syntaxial overgrowths of detrital quartz grains and megaquartz mosaics in remnant porosity (Sqtz).
Some limestone clasts show obliterative diagenetic fabrics and they have been classified here as sparstone clasts. However, the distribution pattern of clots, the size of cavities and the appearance of archaeocyath ghosts suggest that they were calcimicrobe–archaeocyath-bearing microfacies that underwent neomorphism and partial replacement processes that were associated with fracture-related fluids during burial (Fig. 10h, i). In fact, replacive microdolomite crystals occur in the limestone clasts associated with hair-like fractures with ferroan carbonate fluids from the LVB system. This replacive microdolomite is observed in host rock, calcimicrobes, archaeocyaths and shallow marine cements (Fig. 10j, k).
Some samples from the Cambrian Mount Wegener Formation and the Cenozoic erratics show evidence of low-grade tectonically induced fabrics. Furthermore, in the Mount Wegener Formation, the tectonically induced fabrics occur close to the overthrust. Buggisch et al. (Reference Buggisch, Kleinschmidt, Kreuzer and Krumm1994b) described how the sandstones and polymictic conglomerates from the Mount Wegener Formation are tightly folded and deformed above the decollement zone. They observed how the limestone clasts were extremely stretched while feldspars were brittly deformed and described the growth of illite/sericite, chlorite and minor biotite. They recognised two stages of deformation: a first isoclinal folding with penetrative schistosity and distinct crenulation cleavage, and a later recumbent open folding development during the final emplacement of the Mount Wegener Nappe.
We have recognised in the carbonate clasts several features associated with the low-grade tectonically induced fabrics produced during the deformation and emplacement of the Mount Wegener Nappe. In some samples, archaeocyaths and cements can be distorted by the effects of plastic deformation (Fig. 10l), as well as flattened ooids produced by pervasive tectonic shear. Calcite cements associated with late cement veins (LVA and LVB) present different degrees of twin lamellae development. The non-ferroan to very slightly ferroan coarsely poikilotopic calcite cement that precipitated from the LVA system (PkCc in Figs 9d, 10c, h) exhibits mostly type I and rare type II calcite twins. However, the coarse to very coarse crystalline zoned ferroan calcite cement that precipitated from the LVB system exhibits types II (tabular thick) and III (tabular thick curved) calcite twins (Fig. 10d–g). Calcite twin morphologies can be used as a low-temperature geothermometer based on data collection and analysis from Ferrill et al. (Reference Ferrill, Morris, Evans, Burkhard, Groshong and Onasch2004). These authors correlate the average calcite twin with the temperature of deformation, so that the thin twins dominate below 170 °C, and the thick twins dominate above 200 °C. On the other hand, late cement vein systems (LVA and LVB) show the coexistence of calcite and quartz in the veins. Experimental work on calcite and quartz solubility shows that the quartz solubility increases with rising temperatures while the solubility of calcite decreases; between 150 °C and 300 °C, all proportions are possible (Sharp Reference Sharp1965). These temperature values are in agreement with the very low-grade metamorphism conditions reached by the Mount Wegener Formation at the southern Read Mountains according with the illite crystallinities of °Δ2θ > 0.25 (Buggisch et al. Reference Buggisch, Kleinschmidt, Kreuzer and Krumm1994b).
The analysed conglomerates and carbonate clasts may show different degrees of cataclastic deformation (Fig. 5b–e), from minor to dense anastomosing cleavage and incipient brecciation to strongly sheared fabrics (Fig. 10m) with finer seams of solution residues. This deformation post-dates all observed cementation and replacement phases, including late cement veins (LVA and LVB systems; Fig. 10e–g). K–Ar analysis in phyllosilicates (2–6 μm fraction) indicates that low-grade metamorphic overprint and southwards transport of the Mount Wegener Nappe were around 490 Ma (i.e., Furongian) as a result of the Ross (Pan-African) orogeny (Buggisch et al. Reference Buggisch, Kleinschmidt, Kreuzer and Krumm1994b).
7. Systematic palaeontology
Phylum Porifera Grant, Reference Grant and Todd1836
Class Archaeocyatha Bornemann, Reference Bornemann1884
Order Monocyathida Okulitch, Reference Okulitch1935
Family Tumuliolynthidae Rozanov in Rozanov & Missarzhevskiy, Reference Rozanov and Missarzhevskiy1966
Genus Tumuliolynthus Zhuravleva, Reference Zhuravleva1963
Tumuliolynthus irregularis (Bedford & Bedford, Reference Bedford and Bedford1934)
(Fig. 11a, b)
1934 Monocyathus irregularis R. Bedford & W. R. Bedford, p. 2, pl. 1, fig. 2.
1995 Tumuliolynthus irregularis (R. Bedford & W. R. Bedford) – Zhuravlev & Wood, fig. 2a (top).
2020 Tumuliolynthus irregularis (R. Bedford & W. R. Bedford) – Kruse & Debrenne, p. 17, figs 14, 44A, cum syn
Material. Two specimens: MGM-7209X-8; MGM-7213X-5 (see Appendix 2 for localities).

Figure 11 (A, B) Tumuliolynthus irregularis (Bedford & Bedford, Reference Bedford and Bedford1934): (A) MGM-7209X-8, Stephenson Bastion; (B) MGM-7213X-5, Trueman Terraces. (C–E) Dokidocyathus sp.: (C) MGM-7208X-13, Stephenson Bastion; (D) MGM-7209X-17, Stephenson Bastion; (E) MGM-7235X-8, Oldhamia Terraces. (F) Kaltatocyathus gregarius (Gravestock, Reference Gravestock1984): MGM-7218X-29/ 30, Trueman Terraces. (G) Kymbecyathus avius Debrenne & Kruse, Reference Debrenne and Kruse1986: MGM-7249X-2, Stephenson Bastion. Negative. (H) Nochoroicyathus hystrix Kruse, Reference Kruse1982: MGM-7209X-15, Stephenson Bastion. Scale bars = 1 mm (A–F); 2 mm (G, H).
Description. One-walled cup 1.2 to 1.7 mm diameter. The wall is 0.08 to 0.12 mm thick and bears scarce single-pore tumuli with 0.22 to 0.28 mm diameter.
Remarks. This species is characterised by the irregularity of the size and spacing of its tumuli pores.
Occurrence. Australia: Arrowie Basin, Ajax Mine, Ajax Limestone (Bedford & Bedford Reference Bedford and Bedford1934, Reference Bedford and Bedford1939; Zhuravleva Reference Zhuravleva1963; Debrenne Reference Debrenne1969, Reference Debrenne1974b; Kruse & Debrenne Reference Kruse and Debrenne2020); Gnalta Shelf, Mount Wright, Cymbric Vale Formation (Kruse Reference Kruse1982). Allochthonous clasts: South America, Falkland Islands, Hill Cove, Fitzroy Tillite Formation, erratics from the Late Carboniferous (Stone et al. Reference Stone, Thomson and Rushton2012). Antarctica, King George Island, erratic deposits (Zhuravlev & Wood Reference Zhuravlev and Wood1995); King George Island, Admiralty Bay-Melville Peninsula, Oligocene Polonez Cove and Early Miocene Cape Melville Formations, glacio-marine deposits (Wrona & Zhuravlev Reference Wrona and Zhuravlev1996); Shackleton Range, Trueman Terraces, carbonate clasts from the Mount Wegener Formation; Stephenson Bastion, Cenozoic glacial erratic tills. Cambrian Series 2, Botoman.
Order Ajacicyathida Bedford & Bedford, Reference Bedford and Bedford1939
Suborder Dokidocyathina Vologdin, Reference Vologdin1957
Superfamily Dokidocyathoidea Bedford & Bedford, Reference Bedford and Bedford1936
Family Dokidocyathidae Bedford & Bedford, Reference Bedford and Bedford1936
Genus Dokidocyathus Taylor, Reference Taylor1910
Dokidocyathus sp.
(Fig. 11c–e)
Material. Thirteen specimens: MGM-7203X-1, MGM-7206X-1, MGM-7208X-6, MGM-7208X-13; MGM-7208X-15, MGM-7208X-19, MGM-7209X-9, MGM-7209X-17, MGM-7209X-22; MGM-7214X-10; MGM-7235X-8; MGM-7235X-9; MGM-7235X-13 (see Appendix 2 for localities).
Description. Cup 0.7 to 3.8 mm in diameter and intervallum 0.20–1.20 mm wide. Intervallar coefficient IK 0.24–0.40. Outer wall with 3–5 rows of simple pores per intersept (diameter 0.06–0.16 mm, lintels 0.06–0.12 mm, wall thickness 0.04–0.20 mm). Inner wall with 1–2 rows of simple rounded pores per intersept (diameter 0.10–0.20 mm, lintels 0.06–0.20 mm, wall thickness 0.04–0.14 mm). Intervallum traversed by radial bars (thickness 0.02–0.16 mm), the transverse distance of bars 0.18–0.20 mm and longitudinal distance of bars 0.20–0.24 mm.
Remarks. The narrower spacing of intervallar bars and the values of IK distinguish from Dokidocyathus simplicissimus present in Nimrod Glacier (Debrenne & Kruse Reference Debrenne and Kruse1986) and other Australian described species. Although for similar diameters the intervallar bar spacing is in the range of the Australian species zero, the small size of the cups does not allow us to assign them to a specific species.
Occurrence. Allochthonous clasts: Antarctica, Shackleton Range, Trueman Terraces, carbonate clasts from the Mount Wegener Formation; Stephenson Bastion, Cenozoic glacial erratic tills. Cambrian Series 2, Botoman.
Superfamily Kaltatocyathoidea Rozanov in Zhuravleva, Konyushkov & Rozanov, Reference Zhuravleva, Konyushkov and Rozanov1964
Family Kaltatocyathidae Rozanov in Zhuravleva, Konyushkov & Rozanov, Reference Zhuravleva, Konyushkov and Rozanov1964
Genus Kaltatocyathus Rozanov in Zhuravleva, Konyushkov & Rozanov, Reference Zhuravleva, Konyushkov and Rozanov1964
Kaltatocyathus gregarius (Gravestock, Reference Gravestock1984)
(Fig. 11f)
1984 Aroonacyathus gregarius Gravestock, p. 46, fig. 31e–l.
1989 Kaltatocyathus gregarius (Gravestock) – Debrenne, Zhuravlev & Rozanov, p. 114.
1990 Kaltatocyathus gregarius (Gravestock) – Debrenne, Rozanov & Zhuravlev, p. 148.
Material. One specimen: MGM-7218X-29/30 (see Appendix 2 for locality).
Description. Branching cups 1.3–1.6 mm in diameter and intervallum 0.40 mm wide with radial bars 0.02 mm thick. Outer wall with simple tumuli 0.10 mm in height (pore diameter 0.08 mm, wall thickness 0.02 mm). Inner wall with 1–2 rows of simple pores (diameter 0.08 mm, lintels 0.02 mm, wall thickness 0.02–0.04 mm).
Remarks. The porosity of the walls is characteristic of this genus and the branching cups are similar to the transverse section assigned to K. gregarius (Gravestock Reference Gravestock1984, fig. 31g). This is the first recorded occurrence of the Kaltatocyathus from Antarctica.
Occurrence. Australia: Arrowie Basin, Mount Scott Range, Ajax Limestone, Spirillicyathus tenuis Zone (Gravestock Reference Gravestock1984). Allochthonous clasts: Antarctica, Shackleton Range, Trueman Terraces, carbonate clasts from the Mount Wegener Formation. Cambrian Series 2, Botoman. Kaltatocyathus gregarius has been recorded in Australia and correlated with the Atdabanian stage, the type species from Mount Scott Range appears in the Lower Faunal Assemblage II (Gravestock Reference Gravestock1984) or the equivalent Spirillicyathus tenuis Zone (Zhuravlev & Gravestock Reference Zhuravlev and Gravestock1994). In Shackleton Range, K. gregarius occurs with Nochoroicyathus lawrencei, Rotundocyathus glacius, Cadniacyathus sp., Buggischicyathus microporus, Shackletoncyathus buggischi, Paranacyathus sarmaticus and Archaeopharetra irregularis in the same calcimicrobe–archaeocyath boundstone thin section. Therefore, K. gregarius extends its stratigraphic range to the Botoman.
Superfamily Kymbecyathoidea Debrenne, Rozanov & Zhuravlev in Debrenne, Zhuravlev & Rozanov, Reference Debrenne, Zhuravlev and Rozanov1989
Family Kymbecyathidae Debrenne, Rozanov & Zhuravlev in Debrenne, Zhuravlev & Rozanov, Reference Debrenne, Zhuravlev and Rozanov1989
Genus Kymbecyathus Debrenne & Kruse, Reference Debrenne and Kruse1986
Kymbecyathus avius Debrenne & Kruse, Reference Debrenne and Kruse1986
(Fig. 11g)
1986 Kymbecyathus avius Debrenne & Kruse, p. 241, fig. 6.
1989 Kymbecyathus avius Debrenne & Kruse – Debrenne, Zhuravlev & Rozanov, p. 116.
1990 Kymbecyathus avius Debrenne & Kruse – Debrenne, Rozanov & Zhuravlev, p. 149.
2002 Kymbecyathus avius Debrenne & Kruse – Debrenne, Zhuravlev & Kruse, p. 1558, figs 14M, N.
2012 Kymbecyathus avius Debrenne & Kruse – Debrenne, Zhuravlev & Kruse, p. 10, fig. 9.
2015 Kymbecyathus avius Debrenne & Kruse – Debrenne, Zhuravlev & Kruse, p. 932, figs 532a, b.
Material. One specimen: MGM-7249X-2 (see Appendix 2 for locality).
Description. Cup 6.3–8 mm in diameter with intervallum 1.49 mm in width. Outer wall with pore canals (diameter 0.20 mm, lintels 0.12–0.32 mm, wall thickness 0.36 mm). Inner wall with simple, rounded to elliptical pores (diameter 0.20 mm, lintels 0.12 mm, wall thickness 0.12 mm). The presence of possible scattered bars in the intervallum is not confirmed.
Remarks. The diameter of the Byrd Glacier specimens is bigger than specimens described here, but the wall porosity is very similar between all specimens. The bars are rare, scattered and even not visible in some sections (Debrenne & Kruse Reference Debrenne and Kruse1986, fig. 6).
Occurrence. Antarctica: TAM, Byrd Glacier, Crackling Cwm, Shackleton Limestone (Debrenne & Kruse Reference Debrenne and Kruse1986). Allochthonous clasts: Shackleton Range, Stephenson Bastion, Cenozoic glacial erratic tills. Cambrian Series 2, Atdabanian?–Botoman?. In the TAM, the type species from Byrd Glacier was assigned to the Botoman stage (Debrenne & Kruse Reference Debrenne and Kruse1986), but now may possibly be of Atdabanian (Zhuravlev & Gravestock Reference Zhuravlev and Gravestock1994; Kruse & Debrenne Reference Kruse and Debrenne2020). In the Shackleton Range, K. avius occurs with other unidentified specimens; therefore, the age of this sample cannot be clearly specified.
Suborder Ajacicyathina Bedford & Bedford, Reference Bedford and Bedford1939
Superfamily Bronchocyathoidea Bedford & Bedford, Reference Bedford and Bedford1936
Family Ajacicyathidae Bedford & Bedford, Reference Bedford and Bedford1939
Genus Nochoroicyathus Zhuravleva, Reference Zhuravleva1951
Nochoroicyathus hystrix Kruse, Reference Kruse1982
(Fig. 11h)
1982 Nochoroicyathus hystrix Kruse, p. 175–176, pl. 5, figs 1–4.
Material. One specimen: MGM-7209X-15 (see Appendix 2 for locality).
Description. Cup 5.6–6.8 mm in diameter. Intervallum 0.8 mm in width. Radial coefficient RK 7.3–8.9. Intervallar coefficient IK 0.14. Ratio of sides of interseptal loculi IC 1:2.8–1:3.3. Outer wall with 3–4 rows of diaphragm pores per intersept (diameter 0.06 mm, lintels 0.02 mm, wall thickness 0.02 mm). Inner wall with 2–3 rows of simple pores per intersept (diameter 0.08 mm, lintels 0.03–0.08 mm, wall thickness 0.02–0.03 mm) with a short spine projecting inward into central cavity of each pore. Septa with 4–5 rows of rounded pores (diameter 0.10 mm, lintels 0.04 mm, septa thickness 0.02 mm). Tabulae pectinate.
Remarks. The presence of pectinate tabulae and the inner wall with short spines allows us to assign our specimen to the species from Gnalta Shelf.
Occurrence. Australia: Gnalta Shelf, Mount Wright, Cymbric Vale Formation (Kruse Reference Kruse1982). Allochthonous clasts: Antarctica, Shackleton Range, Stephenson Bastion, Cenozoic glacial erratic tills. Cambrian Series 2, Botoman.
Nochoroicyathus lawrencei (Kruse, Reference Kruse1982)
(Fig. 12a, b)
1982 Aldanocyathus lawrencei Kruse, p. 160–161, text-figs 13g–m.
1989 Nochoroicyathus lawrencei (Kruse) – Debrenne, Zhuravlev & Rozanov, p. 120.
1990 Nochoroicyathus lawrencei (Kruse) – Debrenne, Rozanov & Zhuravlev, p. 152.
Material. Twelve specimens: MGM-7202X-3; MGM-7209X-2; MGM-7209X-4; MGM-7209X-13; MGM-7217X-19; MGM-7218X-28; MGM-7219X-6; MGM-7221X-1; MGM-7222X-5; MGM-7235X-7; MGM-7248X-2; MGM-7248X-5 (see Appendix 2 for localities).

Figure 12 (A, B) Nochoroicyathus lawrencei Kruse, Reference Kruse1982: (A) MGM-7209X-13, Stephenson Bastion; (B) MGM-7202X-3, Du Toit Nunatak. (C–F) Rotundocyathus glacius sp. nov.: (C) holotype, MGM-7217X-6; (D) holotype, MGM-7216X-6, Trueman Terraces; (E) MGM-7204X-2, Stephenson Bastion; (F) MGM-7208X-8, Stephenson Bastion. (G) Nochoroicyathus sp.: MGM-7245X-2, Stephenson Bastion. (H, I) Ajacicyathidae gen. et sp. indet: (H) MGM-7239X-5, Oldhamia Terraces; (I) MGM-7238X-3, Oldhamia Terraces. Scale bars = 2 mm (A–E, G, H); 1 mm (F, I).
Description. Cup 1–4.8 mm in diameter. Intervallum 0.3–0.8 mm in width. Radial coefficient RK 3.8–7. Intervallar coefficient IK 0.2–0.4. Ratio of sides of interseptal loculi IC 1:1–1:2.5. Outer wall with 2–5 (8) rows of simple pores per intersept (diameter 0.04–0.14 mm, lintels 0.02–0.10 mm, wall thickness 0.02–0.10 mm). Inner wall with 2–4 rows of simple pores per intersept (diameter 0.08–0.16 mm, lintels 0.02–0.12 mm, wall thickness 0.02–0.10 mm). Septa with 2–5 rows of rounded pores (diameter 0.06–0.16 mm, lintels 0.04–0.12 mm, septa thickness 0.02–0.04 mm).
Remarks. The Shackleton Range specimens are smaller than those described from the Gnalta Shelf and N. cf. lawrencei from Ajax Mine, but the porosity of the walls and septa are similar. The radial and intervallar coefficients vary proportionally during the growth of the cups. The porosity coefficient in both walls is greater in the smaller cups and decreases when the diameter of the cup increases, as observed in Shackleton Range specimens, until it stabilises since it presents little variability from diameters of 4 mm with coefficient values from 1 to 1.5, as observed in N. lawrencei and N. andersoni from Australia. This pattern does not hold for N. cf. lawrencei. The septal porosity coefficient tends to decrease with increasing cup diameter, with values >1, except for some N. lawrencei specimens (~0.6 outer wall Ø/l); this trend is not followed by N. cf. lawrencei.
Occurrence. Australia: Gnalta Shelf, Mount Wright, Cymbric Vale Formation (Kruse Reference Kruse1982). Allochthonous clasts: Antarctica, Shackleton Range, Trueman Terraces, Oldhamia Terraces, Swinnerton Ledge, carbonate clasts from the Mount Wegener Formation; Stephenson Bastion, Du Toit Nunataks, Cenozoic glacial erratic tills. Cambrian Series 2, Botoman.
Nochoroicyathus sp.
(Fig. 12g)
Material. Seven specimens: MGM-7202X-1; MGM-7214X-1; MGM-7214X-3 = MGM-7215X-3 (two different sections of the same specimen); MGM-7227X-9; MGM-7228X-10; MGM-7229X-13; MGM-7245X-2 (see Appendix 2 for localities).
Description. Cup 1.6–9.5 mm in diameter. Intervallum 0.48–1.49 mm in width. Radial coefficient RK 3.3–3.5. Intervallar coefficient IK 0.11–0.30. Ratio of sides of interseptal loculi IC 1:1.7–1:2.8. Outer wall with 2–5 rows of simple pores per intersept (diameter 0.10 mm, lintels 0.06 mm, wall thickness 0.04–0.08 mm). Inner wall with 1–3 rows of simple pores per intersept (diameter 0.12–0.20 mm, lintels 0.04–0.08 mm, wall thickness 0.04–0.08 mm). Septa with 2–6 rows of rounded pores (diameter 0.12 mm, lintels 0.04–0.08 mm, septa thickness 0.04 mm).
Remarks. The poor state of preservation of the cups, affected by recrystallisation and deformation processes, does not allow us to assign them to a described species.
Occurrence. Allochthonous clasts: Antarctica, Shackleton Range, Trueman Terraces, Oldhamia Terraces, carbonate clasts from the Mount Wegener Formation; Stephenson Bastion, Du Toit Nunataks, Cenozoic glacial erratic tills. Cambrian Series 2, Botoman.
Genus Rotundocyathus Vologdin, Reference Vologdin1960
Rotundocyathus glacius Perejón, Menéndez & Moreno-Eiris sp. nov.
(Fig. 12c–f)
Etymology. From Latin ‘glacies’, meaning ‘ice’.
Material. Twenty specimens: MGM-7216X-6 = MGM-7217X-6 (holotype, two different sections of the same specimen); MGM-7210X-6, MGM-7210X-9, MGM-7212X-5, MGM-7214X-2 = MGM-7215X-2, MGM-7218X-40, MGM-7218X-41, MGM-7219X-14 (paratypes); MGM-7204X-1; MGM-7204X-2; MGM-7206X-2; MGM-7208X-8, MGM-7208X-11; MGM-7208X-16; MGM-7208X-21; MGM-7223X-2, MGM-7223X-3, MGM-7223X-4, MGM-7246X-2; MGM-7247X-7 (see Appendix 2 for localities).
Diagnosis. Outer wall with two to six rows of diaphragm pores. Inner wall with one row of simple pores per intersept, without small spines. Septa completely porous, with two to five rows of pores.
Description. Cup 0.8–5.8 mm in diameter. Intervallum 0.3–1.2 mm in width. Radial coefficient RK 2.8–13.9. Intervallar coefficient IK 0.19–0.36. Ratio of sides of interseptal loculi IC 1:1.5–1:4.3. Outer wall with 2–6 rows of diaphragm pores per intersept (diameter 0.06–0.16 mm, lintels 0.02–0.10 mm, wall thickness 0.02–0.20 mm). Inner wall with one row of simple pores per intersept (diameter 0.08–0.24 mm, lintels 0.02–0.12 mm, wall thickness 0.02–0.14 mm). Septa with 2–5 (6) rows of rounded pores (diameter 0.06–0.20 mm, lintels 0.04–0.20 mm, septa thickness 0.02–0.20 mm).
Remarks. Note the existence of endostructures in some specimens and somewhat thickened walls. Some cups have redimiculi (Fig. 12e) at internal and external sides of the outer wall. But they are not similar to Rotundocyathus pelmani Zhuravleva 1997 (Zhuravleva et al. Reference Zhuravleva, Konyaeva, Osadchaya and Boyarinov1997) for having diaphragm pores and greater number of pores in the outer wall, similar cup diameters and absence of pectinate tabulae. It is the first occurrence of this genus in Antarctica, the species Rotundocyathus magnipora was described in Ajax Mine by Kruse & Debrenne (Reference Kruse and Debrenne2020), which differs from the size of the pores of both walls and septa.
Occurrence. Allochthonous clasts: Antarctica, Shackleton Range, Trueman Terraces, Swinnerton Ledge, carbonate clasts from the Mount Wegener Formation; Stephenson Bastion, Cenozoic glacial erratic tills. Cambrian Series 2, Botoman.
Ajacicyathidae gen. et sp. indet.
(Fig. 12h, i)
Material. Twenty-one specimens: MGM-7225X-1; MGM-7227X-4; MGM-7227X-7; MGM-7228X-6; MGM-7228X-9; MGM-7229X-1; MGM-7229X-2; MGM-7229X-3; MGM-7229X-6; MGM-7229X-7; MGM-7229X-8; MGM-7229X-10; MGM-7229X-11; MGM-7229X-12; MGM-7229X-17; MGM-7229X-18; MGM-7235X-19; MGM-7238X-2; MGM-7238X-3; MGM-7239X-5; MGM-7242X-10 (see Appendix 2 for localities).
Remarks. The poor preservation and the fragmentary state of these specimens do not allow us to assign them to a specific genus and species.
Occurrence. Allochthonous clasts: Antarctica, Shackleton Range, Oldhamia Terraces, carbonate clasts from the Mount Wegener Formation. Cambrian Series 2, Botoman.
Family Densocyathidae Vologdin, Reference Vologdin1937
Genus Cadniacyathus Bedford & Bedford, Reference Bedford and Bedford1937
Cadniacyathus sp.
(Fig. 13a, b)
Material. Fifteen specimens: MGM-7210X-5 = MGM-7211X-5; MGM-7216X-2 = MGM-7217X-2; MGM-7216X-7 = MGM-7217X-7; MGM-7216X-8 = MGM-7217X-8; MGM-7216X-14 = MGM-7217X-14; MGM-7218X-31; MGM-7218X-34; MGM-7218X-39; MGM-7219X-10; MGM-7225X-4; MGM-7225X-6; MGM-7227X-10; MGM-7233X-9; MGM-7237X-1 = MGM-7239X-1; MGM-7241X-5 (see Appendix 2 for localities).

Figure 13 (A, B) Cadniacyathus sp.: (A) MGM-7216X-7; Trueman Terraces; (B) MGM-7241X-5, Oldhamia Terraces. (C–E) Buggischicyathus microporus gen. et sp. nov.: (C) MGM-7234X-7, Oldhamia Terraces; (D) holotype, MGM-7210X-3; (E) holotype, MGM-7211X-3, Trueman Terraces. (F–H) Thalamocyathus trachealis (Taylor, Reference Taylor1910): (F) MGM-7208X-18; (G) MGM-7208X-14; (H) MGM-7208X-9, Stephenson Bastion. Scale bars = 2 mm.
Description. Cup 1.8–8.6 mm in diameter. Intervallum 0.5–2.5 mm in width. Radial coefficient RK 2.6–7.7. Intervallar coefficient IK 0.18–0.32. Ratio of sides of interseptal loculi IC 1:1–1:3.7. Outer wall with 2–4 rows of simple pores per intersept (diameter 0.06–0.20 mm, lintels 0.04–0.12 mm, wall thickness 0.02–0.08 mm). Inner wall with 1–3 rows of simple pores per intersept (diameter 0.12–0.48 mm, lintels 0.04–0.20 mm, wall thickness 0.02–0.30 mm); each pore with planar fused bract (length 0.08–0.18 mm). Septa with 2–6 rows of pores (diameter 0.10–0.32 mm, lintels 0.08–0.24 mm, septa thickness 0.02–0.24 mm).
Remarks. The poor state of preservation of many of these specimens and high degree of fragmentation, making many of them incomplete, do not allow us to assign them to some Australian or Antarctic species of Cadniacyathus. There are some differences with the Australian species Cadniacyathus asperatus such as the non-bulging outer wall and its smaller number of pores and the septal porosity.
Occurrence. Allochthonous clasts: Antarctica, Shackleton Range, Trueman Terraces, Oldhamia Terraces, carbonate clasts from the Mount Wegener Formation. Cambrian Series 2, Botoman.
Genus Buggischicyathus Perejón, Menéndez & Moreno-Eiris gen. nov.
Etymology. In memory of Werner Buggisch, a German geologist who sampled and studied this Antarctic material.
Type species. Buggischicyathus microporus Perejón, Menéndez & Moreno-Eiris sp. nov. Cambrian Series 2, Botoman. Shackleton Range, Trueman Terraces, carbonate clast from Mount Wegener Formation.
Diagnosis. Outer wall with several simple pores. Inner wall with one row of pores per intersept, bearing upwardly projecting cupped bracts. Septa with two to six rows of pores, stirrup pores are absent.
Remarks. Buggischicyathus Perejón, Menéndez & Moreno-Eiris differs from Dailycyathus Debrenne (Reference Debrenne1970) by the absence of stirrup pores and the porosity of the septa. From Deceptioncyathus Gravestock (Reference Gravestock1984) for the absence of synapticulae. From Leptosocyathus Vologdin (Reference Vologdin1937) for not having upwardly S scales in the inner wall and the different septal porosity.
Buggischicyathus microporus Perejón, Menéndez & Moreno-Eiris gen. et sp. nov.
(Fig. 13c–e)
Etymology. From Latin ‘microporous’, meaning ‘small pores’.
Material. Seven specimens: MGM-7210X-3 = MGM-7211X-3 (holotype); MGM-7214X-4, MGM-7218X-32 (paratypes); MGM-7229X-14; MGM-7230X-1; MGM-7234X-7; MGM-7236X-1 (see Appendix 2 for localities).
Diagnosis. Cup 2.8–10 mm in diameter. Outer wall with two to four pore rows, inner wall with one pore row per intersept, bearing upwardly projecting cupped bracts. Septa completely porous with rounded to elliptical pores and two to six pore rows.
Description. Cup 2.8–10 mm in diameter. Intervallum 0.80–1.32 mm in width. Radial coefficient RK 6–7.4. Intervallar coefficient IK 0.19–0.36. Ratio of sides of interseptal loculi IC 1:3.7–1:5. Outer wall with 2–4 rows of simple pores per intersept (diameter 0.06–0.16 mm, lintels 0.02–0.08 mm, wall thickness 0.02–0.10 mm). Inner wall one row of simple pores per intersept (diameter 0.14–0.26 mm, lintels 0.04–0.12 mm, wall thickness 0.02–0.10 mm); each pore bearing upwardly cupped bract (length 0.08–0.24 mm). Septa with 2–6 rows of pores (diameter 0.10–0.16 mm, lintels 0.04–0.08 mm, septa thickness 0.02–0.06 mm).
Remarks. The septa of our specimens have numerous pores of small size, unlike the other genera of the Densocyathidae that have only one pore in the inner wall, besides other notable differences, such as Dailycyathus in the septal porosity and absence of stirrup pores; it differs from Deceptioncyathus by not presenting synapticulae and from Leptosocyathus by not having scales on the inner wall.
Occurrence. Allochthonous clasts: Antarctica, Shackleton Range, Trueman Terraces, Oldhamia Terraces, carbonate clasts from the Mount Wegener Formation. Cambrian Series 2, Botoman.
Densocyathidae gen. et. sp. indet.
(Fig. 14a, b)
Material. Ten specimens: MGM-7218X-36; MGM-7224X-5; MGM-7224X-6; MGM-7225X-7; MGM-7227X-8; MGM-7229X-21; MGM-7239X-6; MGM-7239X-7; MGM-7244X-10; MGM-7244X-11 (see Appendix 2 for localities).

Figure 14 (A, B) Densocyathidae gen. et. sp. indet.: (A) MGM-7239X-6; (B) MGM-7227X-8, Oldhamia Terraces. (C) ?Ussuricyathellus sp.: MGM-7207X-1, Stephenson Bastion. (D) ?Baikalocyathus sp.: MGM-7233X-10, Oldhamia Terraces. (E) Paragnaltacyathus hoeflei gen. et sp. nov.: holotype, MGM-7205X-3, Stephenson Bastion. (F, G) Ladaecyathus sp.: (F) MGM-7234X-1; (G) detail, arrow points to the attached microporous sheath, Oldhamia Terraces. Scale bars: 1 mm (A, G); 2 mm (B–F).
Remarks. The poor state of preservation does not allow us to observe clearly the number of pore rows of the internal wall or the type of bracts.
Occurrence. Allochthonous clasts: Antarctica, Shackleton Range, Trueman Terraces, Oldhamia Terraces, Swinnerton Ledge, carbonate clasts from the Mount Wegener Formation; Stephenson Bastion, Cenozoic glacial erratic tills. Cambrian Series 2, Botoman.
Family Bronchocyathidae Bedford & Bedford, Reference Bedford and Bedford1936
Genus Thalamocyathus Gordon, Reference Gordon1920
Thalamocyathus trachealis (Taylor, Reference Taylor1910)
(Fig. 13f–h)
1910 Archaeocyathus trachealis Taylor, p. 125, text-fig. 22, pl. 1, figs 11n–p, pl. 2, fig. 6 left, pl. 3, figs 11a, pl. 5, figs 27–29i, 28–30 g, pl. 6, fig. 31 pars, pl. 8, figs 45–47 (7–8).
2002 Thalamocyathus trachealis (Taylor) – Debrenne, Zhuravlev & Kruse, p. 1567, figs 19a–d.
2012 Thalamocyathus trachealis (Taylor) – Stone, Thomson & Rushton, p. 211, fig. 6c.
2020 Thalamocyathus trachealis (Taylor) – Kruse & Debrenne, p. 42, figs 31a–c, 37, cum syn.
Material. Nine specimens: MGM-7208X-9; MGM-7208X-14; MGM-7208X-17; MGM-7208X-18; MGM-7208X-23; MGM-7209X-5; MGM-7209X-10; MGM-7209X-16; MGM-7209X-19 (see Appendix 2 for localities).
Description. Cup 1.6–7 mm in diameter. Intervallum 0.48–0.96 mm in width. Radial coefficient RK 5.6–12.7. Intervallar coefficient IK 0.18–0.33. Ratio of sides of interseptal loculi IC 1:3–1:6. Outer wall with 1–3 rows of simple and rounded pores per intersept (diameter 0.08–0.10 mm, lintels 0.02–0.08 mm, wall thickness 0.04–0.06 mm), pores with planar diaphragms. Inner wall annulated with one row of pores per intersept (diameter 0.10–0.20 mm, lintels 0.04–0.14 mm, wall thickness 0.04–0.08 and including annuli 0.36 mm); annuli V-shaped. Septa with 2–4 rows of pores (diameter 0.08–0.12 mm, lintels 0.04–0.10 mm, septa thickness 0.04–0.08 mm), the pores are distributed predominantly in the outer half of the septum.
Remarks. The wall and septal porosity are characteristic of T. trachealis, a common species in the Australia–Antarctica province. It differs from Gordonicyathus because the latter presents a greater number of pores in the septa, in cups of similar diameter.
Occurrence. Australia: Arrowie Basin, Ajax Mine (Taylor Reference Taylor1910; Bedford & Bedford Reference Bedford and Bedford1939; Debrenne Reference Debrenne1973; Kruse & Debrenne Reference Kruse and Debrenne2020), Mount Scott Range (Gravestock Reference Gravestock1984); Stansbury Basin, Ardrossan (Taylor Reference Taylor1910; Zhuravlev & Gravestock Reference Zhuravlev and Gravestock1994), Curramulka-Stansbury (Zhuravlev & Gravestock Reference Zhuravlev and Gravestock1994), Cape d'Estaing (Kruse & Moreno-Eiris Reference Kruse and Moreno-Eiris2013). Antarctica: TAM, Holyoake Range, Nimrod Glacier, Shackleton Limestone (Debrenne & Kruse Reference Debrenne and Kruse1986, Reference Debrenne and Kruse1989); Argentina Range, Schneider Hills limestone (Konyushkov & Shulyatin Reference Konyushkov, Shulyatin and Zhuravleva1980). Allochthonous clasts: Africa, main Karoo Basin, Zwartskraal, Dwyka tillites (Debrenne Reference Debrenne1975). South America, Falkland Islands, Fitzroy Tillite Formation (Stone et al. Reference Stone, Thomson and Rushton2012). Antarctica, King George Island, erratic glaciomarine deposits (Morycowa et al. Reference Morycowa, Rubinowski and Tokarski1982, Wrona & Zhuravlev Reference Wrona and Zhuravlev1996); Weddell Sea area (Gordon Reference Gordon1920); Whichaway Nunataks (Hill Reference Hill1965); Shackleton Range, Stephenson Bastion, Cenozoic glacial erratic tills. Cambrian Series 2, Botoman.
Family Ethmocyathidae Debrenne, Reference Debrenne1969
Genus Baikalocyathus Yazmir in Zhuravleva, Reference Zhuravleva1974
?Baikalocyathus sp.
(Fig. 14d)
Material. Two specimens: MGM-7233X-10; MGM-7242X-5 (see Appendix 2 for localities).
Description. Cup 2.6–2.7 mm in diameter. Intervallum 0.9 mm in width. Intervallar coefficient IK 0.32–0.33. Ratio of sides of interseptal loculi IC 1:3.1. Outer wall with several rows of pores per intersept (diameter 0.08–0.14 mm, lintels 0.04 mm, wall thickness 0.06–0.08 mm). Inner wall with one row of downwardly projecting, straight canals per intersept, bearing supplementary bracts on central cavity side forming V-shaped canals (diameter 0.14–0.16 mm, lintels 0.04–0.06 mm, wall thickness 0.04 and canal length 0.10 mm). Septa with two rows of pores (diameter 0.14 mm, lintels 0.08 mm, septa thickness 0.04 mm).
Remarks. The fragmentary nature of the specimens does not allow us to assign a specific species. Several species of Baikalocyathus have been described in Australia. It is the first find of Baikalocyathus in Antarctica.
Occurrence. Allochthonous clasts: Antarctica, Shackleton Range, Oldhamia Terraces, carbonate clasts from the Mount Wegener Formation. Cambrian Series 2, Botoman. Since in Australia it has been recorded during the Atdabanian age, all the species described from Mount Scott Range appear in the Lower Faunal Assemblage II (Gravestock Reference Gravestock1984) or the equivalent Spirillicyathus tenuis Zone (Zhuravlev & Gravestock Reference Zhuravlev and Gravestock1994). Our specimens are found in calcimicrobial boundstone with archaeocyaths together with Cadniacyathus sp., Santelmocyathus santelmoi and Wegenercyathus sexangulae. Therefore, Baikalocyathus extends its stratigraphic range to the Botoman.
Genus Paragnaltacyathus Perejón, Menéndez & Moreno-Eiris gen. nov.
Etymology. From Greek ‘παρά’, meaning similary to Gnaltacyathus Kruse, Reference Kruse1982.
Type species. Paragnaltacyathus hoeflei Perejón, Menéndez & Moreno-Eiris sp. nov. Cambrian Series 2, Botoman. Shackleton Range, Stephenson Bastion, Cenozoic glacial erratic tills.
Diagnosis. Outer wall with simple pores. Inner wall with straight canals, each canal span several intersepts. Septa completely porous, linked by synapticulae.
Remarks. This genus differs from Gnaltacyathus Kruse (Reference Kruse1982) by presenting synapticulae.
Paragnaltacyathus hoeflei Perejón, Menéndez & Moreno-Eiris gen. et sp. nov.
(Fig. 14e)
Etymology. In memory of Hans-Christian Höfle, German glaciologist who sampled and studied the Cenozoic erratics of all this Antarctic material.
Material. One specimen: MGM-7205X-3 (see Appendix 2 for locality).
Diagnosis. Outer wall with two rows of simple pores. Inner wall with straight canals, each canal span several intersepts. Septa completely porous with five or more rows of pores. Synapticulae.
Description. A fragmented cup. Outer wall with two rows of pores per intersept (diameter 0.20 mm, lintels 0.28 mm, wall thickness 0.32 mm). Inner wall with straight canals, each canal spanning several (2–3) intersepts (diameter 1.10 mm, lintels 0.25 mm, wall thickness 1.50 mm). Septa with five or more rows of pores (diameter 0.50 mm, lintels 0.25 mm, septa thickness 0.17 mm). Sinapticulae thickness 0.17 mm.
Remarks. Although the cup is fragmented, it is possible to recognise the internal wall so characteristic of Gnaltacyathus, but the presence of synapticulae suggests that it is a different genus.
Occurrence. Allochthonous clasts: Antarctica, Shackleton Range, Stephenson Bastion, Cenozoic glacial erratic tills. Cambrian Series 2, Botoman.
Genus Ussuricyathellus Voronin, Reference Voronin1988
?Ussuricyathellus sp.
(Fig. 14c)
Material. One specimen: MGM-7207X-1 (see Appendix 2 for locality).
Description. Cup 7.5 mm in diameter. Intervallum 1.7 mm in width. Intervallar coefficient IK 0.22. Ratio of sides of interseptal loculi IC 1:2.9. Outer wall with several rows of pores per intersept (diameter 0.16 mm, lintels 0.16 mm, wall thickness 0.16 mm). Inner wall with two rows of horizontal to upwardly projecting, straight canals per intersept (diameter 0.20 mm, lintels 0.12–0.32 mm, wall thickness 0.32). Septa with one row of pores or aporose.
Remarks. The porosity of the inner wall and the aporose to sparsely porous septa allow us to assign it to the genus Ussuricyathellus Voronin (Reference Voronin1988). This assumes that it is the first record of this genus in Antarctica. Australian species Ussuricyathellus bellidoi (Kruse & Moreno-Eiris Reference Kruse and Moreno-Eiris2013) and Ussuricyathellus coronus (Kruse & Debrenne Reference Kruse and Debrenne2020) are correlated with the Botoman.
Occurrence. Allochthonous clasts: Antarctica, Shackleton Range, Stephenson Bastion, Cenozoic glacial erratic tills. Cambrian Series 2, Botoman.
Superfamily Erbocyathoidea Vologdin & Zhuravleva in Vologdin, Reference Vologdin1956
Family Erbocyathidae Vologdin & Zhuravleva in Vologdin, Reference Vologdin1956
Genus Ladaecyathus Zhuravleva, Reference Zhuravleva1960a
Ladaecyathus sp.
(Fig. 14f, g)
Material. Four specimens: MGM-7208X-4; MGM-7234X-1; MGM-7241X-6; MGM-7245X-3 (see Appendix 2 for localities).
Description. Cup 5.2–10 mm in diameter. Intervallum 1.2–2.7 mm in width. Intervallar coefficient IK 0.22–0.23. Ratio of sides of interseptal loculi IC 1:2.6–1:5. Outer wall with 2–3 rows of pores per intersept (diameter 0.20–0.58 mm, lintels 0.08–0.58 mm, wall thickness 0.08–0.58 mm); the attached microporous sheath has several micropores (diameter 0.12 mm, lintels 0.04 mm). Inner wall with two rows of simple pores per intersept (diameter 0.18–0.28 mm, lintels 0.08–0.12 mm, wall thickness 0.06–0.16 mm). Septa with 4–6 rows of pores (diameter 0.16–0.36 mm, lintels 0.04–0.20 mm, septa thickness 0.04–0.08 mm).
Remarks. Ladaecyathus jagoi Debrenne & Kruse (Reference Debrenne and Kruse1986) has been described in Nimrod Glacier, but the bad preservation of our material does not allow us to assign it to a specific species.
Occurrence. Allochthonous clasts: Antarctica, Shackleton Range, Oldhamia Terraces, carbonate clasts from the Mount Wegener Formation; Stephenson Bastion, Cenozoic glacial erratic tills. Cambrian Series 2, Botoman.
Superfamily Lenocyathoidea Zhuravleva in Vologdin, Reference Vologdin1956
Family Shackletoncyathidae Perejón, Menéndez & Moreno-Eiris fam. nov.
Etymology. From Shackleton Range.
Diagnosis. Inner wall with bracts or scales.
Generic composition. Two new genera are included: Shackletoncyathus and Santelmocyathus.
Genus Shackletoncyathus Perejón, Menéndez & Moreno-Eiris gen. nov.
Etymology. From Shackleton Range.
Type species. Shackletoncyathus buggischi Perejón, Menéndez & Moreno-Eiris sp. nov. Cambrian Series 2, Botoman. Shackleton Range, Trueman Terraces, carbonate clast from the Mount Wegener Formation.
Diagnosis. Outer wall with multiperforated tumuli. Inner wall with several rows of pores per intersept, bearing supplementary bracts on central cavity side. Septa completely porous.
Remarks. This genus is characterised by inner wall with several rows of pores per intersept, bearing supplementary tubular bracts on central cavity side. Septa completely porous.
Shackletoncyathus buggischi Perejón, Menéndez & Moreno-Eiris gen. et sp. nov.
(Figs 15d, 16)
Etymology. In memory of Werner Buggisch, German geologist who sampled and studied this Antarctic material.

Figure 15 (A, B) Santelmocyathus santelmoi gen. et sp. nov.: (A) holotype, MGM-7246X-4, Stephenson Bastion; (B) MGM-7231X-2, Oldhamia Terraces. (C) Coscinoptycta convoluta (Taylor, Reference Taylor1910): MGM-7216X-5, Trueman Terraces. (D) Shackletoncyathus buggischi gen. et sp. nov.: holotype, MGM-7248X-3, Stephenson Bastion. (E) ?Fallocyathus sp.: MGM-7234X-9, Oldhamia Terraces. Scale bars = 2 mm (A–C); 1 mm (D, E).

Figure 16 3D reconstruction of Shackletoncyathus buggischi (designed by F. Cebrián).
Material. Five specimens: MGM-7248X-3 (Holotype); MGM-7243X-7, MGM-7246X-1 (Paratypes); MGM-7202X-4; MGM-7218X-38 (see Appendix 2 for localities).
Diagnosis. Cup 0.8–3.2 mm in diameter. Outer wall with two to three rows of multiperforated tumuli per intersept. Inner wall with two to three rows of pores per intersept, bearing supplementary tubular bracts on central cavity side. Septa completely porous.
Description. Cup 0.8–3.2 mm in diameter. Intervallum 0.48–0.72 mm in width. Radial coefficient RK 3.1–7.6. Intervallar coefficient IK 0.26. Ratio of sides of interseptal loculi IC 1:1.1–1:2.4. Outer wall with 2–3 rows of multiperforated tumuli per intersept (diameter 0.08–0.12 mm, lintels 0.06 mm, wall thickness 0.02–0.04 mm, tumuli high 0.20 mm). Inner wall with 2–3 rows of pores per intersept (diameter 0.06–0.20 mm, lintels 0.08–0.40 mm, wall thickness 0.02–0.10 mm); each pore bearing supplementary tubular bracts on central cavity side (length up 0.14 mm). Septa with 2–3 (5) rows of pores (diameter 0.10 mm, lintels 0.04–0.06 mm, septa thickness 0.02–0.04 mm).
Remarks. The porosity of the inner wall and the septa are the distinctive character to distinguish our material from the other genera belonging to the other families of the Lenocyathoidea.
Occurrence. Allochthonous clasts: Antarctica, Shackleton Range, Trueman Terraces, carbonate clasts from the Mount Wegener Formation; Stephenson Bastion, Du Toit Nunataks, Cenozoic glacial erratic tills. Cambrian Series 2, Botoman.
Genus Santelmocyathus Perejón, Menéndez & Moreno-Eiris gen. nov.
Etymology. From the San Telmo Spanish ship, possibly the first to reach the coast of the Antarctic continent in 1819.
Type species. Santelmocyathus santelmoi Perejón, Menéndez & Moreno-Eiris sp. nov. Cambrian Series 2, Botoman. Shackleton Range, Trueman Terraces, Oldhamia Terraces, carbonate clasts from the Mount Wegener Formation; Stephenson Bastion, Cenozoic glacial erratic tills. Cambrian Series 2, Botoman.
Diagnosis. Outer wall with multiperforated tumuli. Inner wall with several rows of pores per intersept, bearing possibly upwardly projecting, S-shaped scales. Septa aporose to sparsely porous.
Remarks. This genus is characterised by inner wall with one to two rows of pores per intersept, bearing S-shaped scales. Septa aporose to sparsely porous.
Santelmocyathus santelmoi Perejón, Menéndez & Moreno-Eiris gen. et sp. nov.
(Fig. 15a, b)
Etymology. From the San Telmo Spanish ship, possibly the first to reach the coast of the Antarctic continent in 1819.
Material. Five specimens: MGM-7246X-4 (holotype); MGM-7246X-3 (paratype); MGM-7219X-8; MGM-7231X-2 = MGM-7232X-2; MGM-7242X-2 (see Appendix 2 for localities).
Diagnosis. Cup 2.8–8.3 mm in diameter. Outer wall with one to three rows of multiperforate tumuli. Inner wall with one to two rows of pores per intersept, bearing possibly upwardly projecting, S-shaped scales. Septa aporose to sparsely porous with one to three rows of pores. Radial coefficient RK 2.9–3.8.
Description. Cup 2.8–8.3 mm in diameter. Intervallum 0.5–1.7 mm in width. Radial coefficient RK 2.9–3.8. Intervallar coefficient IK 0.2–0.3. Ratio of sides of interseptal loculi IC 1:1.6–1:3.2. Outer wall with 1–3 rows of multiperforate tumuli per intersept (diameter 0.04–0.28 mm, lintels 0.04–0.20 mm, wall thickness 0.02–0.20 mm, tumuli high 0.14 mm). Inner wall with 1–2 rows of pores per intersept (diameter 0.16–0.24 mm, lintels 0.04–0.16 mm, wall thickness 0.04–0.30 mm); each pore bearing possibly upwardly projecting, S-shaped scales (length 0.12 mm). Septa with 1–3 rows of pores (diameter 0.08–0.12 mm, lintels 0.04–0.16 mm, septa thickness 0.04–0.24 mm).
Remarks. Santelmocyathus santelmoi differs from Shackletoncyathus buggischi in the inner wall, by having scales and not bracts, and the septal porosity, as it occurs in another new genus of family Shackletoncyathidae.
Occurrence. Allochthonous clasts: Antarctica, Shackleton Range, Trueman Terraces, Oldhamia Terraces, carbonate clasts from the Mount Wegener Formation; Stephenson Bastion, Cenozoic glacial erratic tills. Cambrian Series 2, Botoman.
Superfamily Ethmophylloidea Okulitch, Reference Okulitch1937
Family Fallocyathidae Rozanov in Zhuravleva, Korshunov & Rozanov, Reference Zhuravleva, Korshunov, Rozanov and Zhuravleva1969
Genus Fallocyathus Rozanov in Zhuravleva, Korshunov & Rozanov, Reference Zhuravleva, Korshunov, Rozanov and Zhuravleva1969
?Fallocyathus sp.
(Fig. 15e)
Material. Two specimens: MGM-7234X-8 and MGM-7234X-9 (see Appendix 2 for localities).
Description. Cup 1–5.8 mm in diameter. Intervallum 0.5–1 mm in width. Radial coefficient RK 3.5. Intervallar coefficient IK 0.2–0.3. Ratio of sides of interseptal loculi IC 1:1.3–1:1.5. Outer wall with 3–4 rows of canals per intersept (diameter 0.10 mm, lintels 0.06–0.12 mm, wall thickness 0.10–0.20 mm). Inner wall with 2–3 rows of simple pores per intersept (diameter 0.14–0.16 mm, lintels 0.04–0.80 mm, wall thickness 0.04–0.06 mm). Septa with 1–3 rows of pores (diameter 0.10–0.16 mm, lintels 0.04–0.08 mm, septa thickness 0.04 mm).
Remarks. The canals of the outer wall and the simple pores of the inner wall are characteristic of Fallocyathus. The type of preservation of cup sections does not allow us to assign them to a specific species.
Occurrence. Allochthonous clasts: Antarctica, Shackleton Range, Oldhamia Terraces, carbonate clasts from the Mount Wegener Formation. Cambrian Series 2, Botoman.
Suborder Erismacoscinina Debrenne, Rozanov & Zhuravlev in Debrenne, Zhuravlev & Rozanov, Reference Debrenne, Zhuravlev and Rozanov1989
Superfamily Salairocyathoidea Zhuravleva in Vologdin, Reference Vologdin1956
Family Asterocyathidae Vologdin, Reference Vologdin1956
Genus Antoniocoscinus Zhuravlev in Debrenne et al., Reference Debrenne, Zhuravlev and Rozanov1988
?Antoniocoscinus sp.
(Fig. 17b)
Material. Two specimens: MGM-7227X-3; MGM-7231X-3 = MGM-7232X-3 (see Appendix 2 for localities).
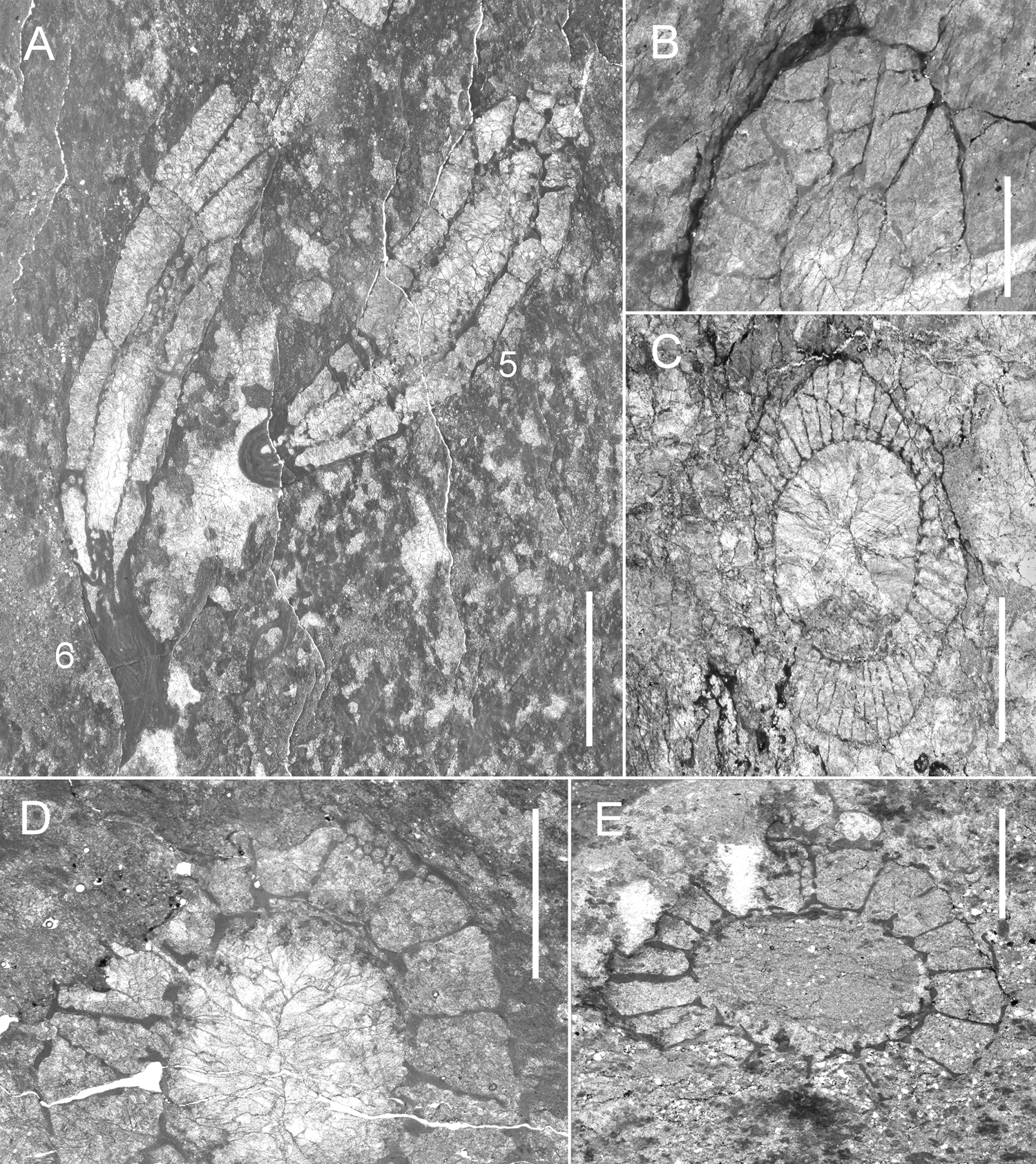
Figure 17 (A) Erismacoscinus bilateralis (Taylor, Reference Taylor1910): MGM-7232X-5 and 6, Oldhamia Terraces. (B) ?Antoniocoscinus sp.: MGM-7231X-3, Oldhamia Terraces. (C) ?Retecoscinus sp.: MGM-7227X-12, Oldhamia Terraces. (D, E) Wegenercyathus sexangulae gen. et sp. nov.: (D) holotype, MGM-7233X-11; (E) paratype, MGM-7242X-6, Oldhamia Terraces. Scale bars = 4 mm (A); 2 mm (B–E).
Description. Cup 1.4–8.3 mm in diameter. Intervallum 0.52–0.80 mm in width. Radial coefficient RK 2.9–12.5. Intervallar coefficient IK 0.30–0.36. Ratio of sides of interseptal loculi IC 1:2.8–1:4.3. Outer wall with 2–3 rows of simple pores per intersept (diameter 0.06 mm, lintels 0.02–0.04 mm, wall thickness 0.02–0.08 mm). Inner wall with one row of simple pores per intersept (diameter 0.28 mm, lintels 0.16 mm, wall thickness 0.04 mm). Septa with three? rows of pores (diameter 0.04 mm, lintels 0.02 mm, septa thickness 0.02–0.04 mm). Tabulae 0.04 in thickness, with 3–4 rows of pores per loculus (diameter 0.10 mm, lintels 0.06 mm).
Remarks. The specimens are intensely affected by recrystallisation and tectonic deformation processes, which does not allow assigning them to a specific species. Antoniocoscinus retifer is present in Ajax Mine.
Occurrence. Allochthonous clasts: Antarctica, Shackleton Range, Oldhamia Terraces, carbonate clasts from the Mount Wegener Formation. Cambrian Series 2, Botoman.
Genus Erismacoscinus Debrenne, Reference Debrenne1958
Erismacoscinus bilateralis (Taylor, Reference Taylor1910)
(Fig. 17a)
1910 Coscinoptycha bilateralis Taylor, p. 142, text-fig. 6, pl. 2, fig. 6, pl. 6, fig. 32, pl. 11, figs 61–63.
2020 Erismacoscinus bilateralis (Taylor) – Kruse & Debrenne, p. 76, figs 65, 66, cum syn.
Material. Two specimens: MGM-7232X-5 and MGM-7232X-6 (see Appendix 2 for localities).
Description. Conical cup 3–5.5 mm in diameter. Intervallum 0.9–1.1 mm in width. Intervallar coefficient IK 0.20–0.29. Ratio of sides of interseptal loculi IC 1:1.4–1:3.6. Outer wall with several rows of simple pores per intersept (diameter 0.16 mm, lintels 0.16 mm, wall thickness 0.04–0.12 mm). Inner wall with 1–2 rows of simple pores per intersept (diameter 0.16–0.28 mm, lintels 0.04–0. 12 mm, wall thickness 0.04–0.28 mm). Septa with 2–3 rows of pores (diameter 0.16–0.20 mm, lintels 0.08–0.12 mm, septa thickness 0.04–0.12 mm). Tabulae spacing 1–1.6 mm, with 3–6 radial rows of pores (diameter 0.06–0.12 mm, lintels 0.02–0.08 mm, thickness 0.02–0.04 mm).
Remarks. Measurements and coefficients of Shackleton specimens fall within the range of the species Erismacoscinus bilateralis described by Kruse & Debrenne (Reference Kruse and Debrenne2020), which includes some specimens of Gordon's Reference Gordon1920 E. endutus and E. fultus from Weddell Sea.
Occurrence. Australia: Amadeus and Georgina Basins (Kruse & West Reference Kruse and West1980); Arrowie Basin, Ajax Mine, Ajax Limestone (Taylor Reference Taylor1910; Kruse & Debrenne Reference Kruse and Debrenne2020); Stansbury Basin, Cape d'Estaing, White Point Conglomerate (Kruse & Moreno-Eiris Reference Kruse and Moreno-Eiris2013). Allochthonous clasts: Antarctica, Weddell Sea area (Gordon Reference Gordon1920); Whichaway Nunataks (Hill Reference Hill1965); Shackleton Range, Oldhamia Terraces, carbonate clasts from the Mount Wegener Formation. Cambrian Series 2, Botoman.
Genus Retecoscinus Zhuravleva Reference Zhuravleva1960b
?Retecoscinus sp.
(Fig. 17c)
Material. One specimen: MGM-7227X-12 (see Appendix 2 for locality).
Description. Cup 3.2–5.6 mm in diameter. Intervallum 0.60 mm in width. Radial coefficient RK 13.8. Intervallar coefficient IK 0.19. Ratio of sides of interseptal loculi IC 1:3.7. Outer wall with 3–4 rows of simple pores per intersept (diameter 0.06 mm, lintels 0.02 mm, wall thickness 0.02 mm). Inner wall with 1–2 rows of simple pores per intersept (diameter 0.14 mm, lintels 0.06 mm, wall thickness 0.04 mm). Septa with 4–6 rows of pores (diameter 0.06 mm, lintels 0.04–0.08 mm, septa thickness 0.02 mm). Tabulae with two slit-like pores per intersept.
Remarks. The porosity of both walls is similar to Retecoscinus apart from the presence of tabulae with slit-like pores, but the designation is doubtful due to the poor preservation of this specimen.
Occurrence. Allochthonous clasts: Antarctica, Shackleton Range, Oldhamia Terraces, carbonate clasts from the Mount Wegener Formation. Cambrian Series 2, Botoman.
Family Rudanulidae Debrenne, Rozanov & Zhuravlev in Debrenne, Zhuravlev & Rozanov, Reference Debrenne, Zhuravlev and Rozanov1989
Genus Wegenercyathus Perejón, Menéndez & Moreno-Eiris gen. nov.
Etymology. After Mount Wegener Formation from Shackleton Range.
Type species. Wegenercyathus sexangulae Perejón, Menéndez & Moreno-Eiris sp. nov. Cambrian Series 2, Botoman. Shackleton Range, Oldhamia Terraces, carbonate clasts from Mount Wegener Formation.
Diagnosis. Outer wall with normal pores. Inner wall with several rows of pores per intersept, bearing S-shaped bracts; septa completely porous; retiform tabulae with subpolygonal pores.
Remarks. Wegenercyathus shares the same septal porosity as Rudanulus, the difference is that Rudanulus has a longitudinally plicate outer wall and inner wall with scales. The new genus differs from Pilodicoscinus in that the latter has plicate outer wall, cupped inner wall bracts and aporose to sparsely porous septa. Wegenercyathus shares the same septal porosity as Yhecyathus, the difference is that the latter has plicate outer wall, and cupped inner wall bracts.
Wegenercyathus sexangulae Perejón, Menéndez & Moreno-Eiris gen. et sp. nov.
(Fig. 17d, e)
Etymology. From Latin ‘sexangulae’, meaning ‘hexagonal pores’.
Material. Two specimens: MGM-7233X-11 (holotype); MGM-7242X-6 (paratype) (see Appendix 2 for localities).
Diagnosis. Outer wall with two or three rows of simple pores per intersept. Inner wall with several rows of pores per intersept, bearing S-shaped bracts; septa completely porous; retiform tabulae with hexagonal pores.
Description. Cup 5.2–7.5 mm in diameter. Intervallum 1.2–1.4 mm in width. Radial coefficient RK 2.3–3.1. Intervallar coefficient IK 0.23–0.26. Ratio of sides of interseptal loculi IC 1:1.5–1:2.8. Outer wall with 2–3 rows of normal pores per intersept (diameter 0.20 mm, lintels 0.20 mm, wall thickness 0.02–0.08 mm). Inner wall with 1–2 rows of pores per intersept (diameter 0.20 mm, lintels 0.08–0.20 mm, wall thickness 0.04–0.08 mm); each pore bearing S-shaped bract (length 0.10–0.20 mm). Septa with 4–6 rows of pores (diameter 0.06–0.08 mm, lintels 0.10 mm, septa thickness 0.04–0.08 mm). Tabulae with 5–6 large hexagonal pores per intersept (diameter 0.14–0.16 mm, lintels 0.04 mm, thickness 0.02–0.04 mm).
Remarks. In addition to the differences already indicated with the other genera of Rudanulidae, the presence of retiform tabulae with large subpolygonal pores distinguishes it, since these hexagonal pores are unusual.
Occurrence. Allochthonous clasts: Antarctica, Shackleton Range, Oldhamia Terraces, carbonate clasts from the Mount Wegener Formation. Cambrian Series 2, Botoman.
Superfamily Coscinoptyctoidea Debrenne, Rozanov & Zhuravlev in Debrenne, Zhuravlev & Rozanov, Reference Debrenne, Zhuravlev and Rozanov1989
Family Coscinoptyctidae Debrenne, Rozanov & Zhuravlev in Debrenne, Zhuravlev & Rozanov, Reference Debrenne, Zhuravlev and Rozanov1989
Genus Coscinoptycta Broili, Reference Broili and Von Zittel1915
Coscinoptycta convoluta (Taylor, Reference Taylor1910)
(Fig. 15c)
1910 Coscinoptycha convoluta Taylor, p. 141, text-figs 7, 8, 33, pl. 11, fig. 60.
2020 Coscinoptycta convoluta (Taylor) – Kruse & Debrenne, p. 102, fig. 87, cum syn.
Material. Two specimens: MGM-7214X-5; MGM-7216X-5 = MGM-7217X-5 (see Appendix 2 for localities).
Description. Cup in which both walls show slight synchronous transverse folds. Diameter 3.6–4.4 mm. Intervallum 0.8–1.2 mm in width. Radial coefficient RK 5.8–5.9. Intervallar coefficient IK 0.2–0.3. Ratio of sides of interseptal loculi IC 1:2.5–1:3. Outer wall with 1–2 rows of pores per intersept (diameter 0.24–0.28 mm, lintels 0.14 mm, wall thickness 0.08 mm), each surmounted by a multiperforate tumulus. Tumuli 0.2–0.3 mm high. Inner wall with 1–3 rows of pores (diameter 0.20 mm, lintels 0.08 mm, wall thickness 0.08 mm), bearing cupped bracts. Septa with 1–3 rows of pores (diameter 0.12 mm, lintels 0.16 mm, septa thickness 0.04–0.08 mm). Tabulae with 3–5 radial rows of pores, tabulae thickness 0.07 mm.
Remarks. The porosity of both walls is characteristic of C. convoluta, including the other species described for the genus that are currently synonymous, which allows us to assign our specimens.
Occurrence. Australia: Arrowie Basin, Ajax Mine (Taylor Reference Taylor1910; Bedford & Bedford Reference Bedford and Bedford1934; Ting Reference Ting1937; Debrenne & Debrenne Reference Debrenne and Debrenne1960; Debrenne Reference Debrenne1969; Kruse & Debrenne Reference Kruse and Debrenne2020), Wirrealpa Mine (Zhuravlev & Gravestock Reference Zhuravlev and Gravestock1994); Mount Scott Range (Gravestock Reference Gravestock1984); Gnalta Shelf, Mount Wright (Kruse Reference Kruse1982); Stansbury Basin, Curramulka-Stansbury (Zhuravlev & Gravestock Reference Zhuravlev and Gravestock1994), Cape d'Estaing (Kruse & Moreno-Eiris Reference Kruse and Moreno-Eiris2013). Allochthonous clasts: Antarctica, Beardmore Glacier (Hill Reference Hill and Adie1964a); Shackleton Range, Trueman Terraces, carbonate clasts from the Mount Wegener Formation. Cambrian Series 2, Botoman.
Order Putapacyathida Vologdin, Reference Vologdin1961
Superfamily Putapacyathoidea Bedford & Bedford, Reference Bedford and Bedford1936
Family Putapacyathidae Bedford & Bedford, Reference Bedford and Bedford1936
Genus Putapacyathus Bedford & Bedford, Reference Bedford and Bedford1936
Putapacyathus sp.
(Fig. 18a)
Material. One specimen: MGM-7220X-4 (see Appendix 2 for locality).

Figure 18 (A) Putapacyathus sp.: MGM-7220X-4, Swinnerton Ledge. (B, C) Neoloculicyathus sp.: (B) MGM-7203X-2; (C) MGM-7208X-1, Stephenson Bastion. (D) Paranacyathus sarmaticus Debrenne, 1974: MGM-7217X-1, arrow indicates diaphragm pores, Trueman Terraces. (E, H) Archaeopharetra irregularis (Taylor, Reference Taylor1910): (E) MGM-7225X-3, Oldhamia Terraces; (H) MGM-7218X-27, Trueman Terraces. (F) Loculicyathidae gen. et sp. indet.: MGM-7242X-8, Oldhamia Terraces. (G) ?Graphoscyphia sp.: MGM-7209X-1, Stephenson Bastion. Scale bars = 4 mm (A, D); 1 mm (B, C, F); 2 mm (E, G, H).
Description. Cup narrowly conical, diameter 6–7 mm with intervallum 1–1.5 mm width. Intervallar coefficient IK 0.16–0.21. Outer wall secondarily thickened, with ten pores per intertabulum (diameter 0.08 mm, lintels 0.04–0.20 mm, wall thickness 0.12 mm). The presence of the microporous sheath is not clearly observed, although redimiculi and vesicular tissue are present. Inner wall with 3–8 rows of pores per intertabulum (diameter 0.10 mm, lintels 0.02 mm, wall thickness 0.10 mm); each pore bearing cupped bract (length 0.12 mm) projects downward. Tabulae flat with 4–8 pores across intervallum (diameter 0.10 mm, lintels 0.04 mm, thickness 0.04 mm).
Remarks. The poor state of preservation of the specimen does not allow the observation of all the diagnostic characters to be able to assign it to a described species, although our material may have slightly wider intervallum than Putapacyathus regularis does, but it is a small difference, and it is found on trend lines of the intervallar coefficient.
Occurrence. Allochthonous clasts: Antarctica, Shackleton Range, Swinnerton Ledge, carbonate clasts from the Mount Wegener Formation. Cambrian Series 2, Botoman.
Order Archaeocyathida Okulitch, Reference Okulitch1935
Suborder Loculicyathina Zhuravleva, Reference Zhuravleva1955
Superfamily Loculicyathoidea Zhuravleva, Reference Zhuravleva1954
Family Loculicyathidae Zhuravleva, Reference Zhuravleva1954
Genus Neoloculicyathus Voronin, Reference Voronin, Zhuravleva and Rozanov1974
Neoloculicyathus sp.
(Fig. 18b, c)
Material. Six specimens: MGM-7202X-2; MGM-7203X-2; MGM-7204X-5; MGM-7207X-2; MGM-7208X-1; MGM-7248X-1 (see Appendix 2 for localities).
Description. Cup conical, diameter 1.7–3.3 mm with intervallum 0.5–0.9 mm wide. Radial coefficient RK 3.7–4.7. Intervallar coefficient IK 0.3–0.4. Ratio of sides of interseptal loculi IC 1:2–1:2.6. Outer wall with 2–3 rows of cambroid pores per intersept (diameter 0. 08–0.12 mm, lintels 0.02–0.08 mm, wall thickness 0.02–0.40 mm). Inner wall with 1–2 (3?) rows of pores per intersept (diameter 0.06–0.20 mm, lintels 0.02–0.16 mm, wall thickness 0.02–0.12 mm). Pseudosepta with 1–5 rows of pores (diameter 0.10–0.18 mm, lintels 0.04–0.08 mm, thickness 0.04–0.06 mm).
Remarks. The poor preservation of our material does not allow us to assign it to a specific species. It is the first occurrence of this genus in Antarctica.
Occurrence. Allochthonous clasts: Antarctica, Shackleton Range, Stephenson Bastion, Du Toit Nunataks, Cenozoic glacial erratic tills. Cambrian Series 2, Botoman.
Genus Paranacyathus Bedford & Bedford, Reference Bedford and Bedford1937
Paranacyathus sarmaticus Debrenne, Reference Debrenne, Zhuravleva and Rozanov1974c
(Fig. 18d)
Reference Bedford and Bedford1937 Paranacyathus parvus Bedford & Bedford (part), p. 34, pl. 35, figs 137a–g.
Reference Debrenne, Zhuravleva and Rozanov1974c Paranacyathus sarmaticus Debrenne, p. 171, pl. 19, figs 5–7.
Reference Debrenne and Zhuravlev1992 Paranacyathus sarmaticus Debrenne & Zhuravlev, p. 128, 145.
Reference Wrona and Zhuravlev1996 Paranacyathus sarmaticus Debrenne – Wrona & Zhuravlev, p. 27, pl. 6, figs 1, 2.
Material. Three specimens: MGM-7210X-4; MGM-7216X-1 = MGM-7217X-1 = MGM-7218X-1 (three different sections of the same specimen); MGM-7218X-35 (see Appendix 2 for localities).
Description. Cup 1.6–9.9 mm in diameter. Intervallum 0.6–2.9 mm in width. Radial coefficient RK 2–3.2. Intervallar coefficient IK 0.3–0.4. Ratio of sides of interseptal loculi IC 1:4.1–1:5.8. Outer wall with two rows of cambroid pores per intersept (diameter 0.20 mm, lintels 0.16 mm, wall thickness 0.12 mm). This wall is slightly bulged over pores producing diaphragms. Inner wall with one (two?) rows of pores per intersept (diameter 0.10–0.40 mm, lintels 0.06–0.08 mm, wall thickness 0.04–0.08 mm). Pseudosepta with 3–6 rows of pores (diameter 0.08–0.20 mm, lintels 0. 80 mm, thickness 0.08 mm).
Remarks. The difference between P. parvus and P. sarmaticus is the absence or presence, respectively, of diaphragms over the outer wall pores. The Shackleton Range specimens present exostructures especially developed in the apical part. Vesicular tissue occupies the intervallum and central cavity in some cups, as well as the specimens described by Wrona & Zhuravlev (Reference Wrona and Zhuravlev1996) from King George Island.
Occurrence. Australia: Arrowie Basin, Ajax Mine, Ajax Limestone (Bedford & Bedford Reference Bedford and Bedford1937; Debrenne Reference Debrenne, Zhuravleva and Rozanov1974c). Allochthonous clasts: Antarctica, King George Island, Admiralty Bay-Melville Peninsula, Oligocene Polonez Cove and Early Miocene Cape Melville Formations, glacio-marine deposits (Wrona & Zhuravlev Reference Wrona and Zhuravlev1996); Shackleton Range, Trueman Terraces, carbonate clasts from the Mount Wegener Formation. Cambrian Series 2, Botoman.
Loculicyathidae gen. et sp. indet.
(Fig. 18f)
Material. Three specimens: MGM-7231X-4; MGM-7242X-3; MGM-7242X-8 (see Appendix 2 for localities).
Remarks. The poor preservation and small size of our material do not allow us to assign it to a specific species.
Occurrence. Allochthonous clasts: Antarctica, Shackleton Range, Oldhamia Terraces, carbonate clasts from the Mount Wegener Formation. Cambrian Series 2, Botoman.
Suborder Archaeocyathina Okulitch, Reference Okulitch1935
Superfamily Dictyocyathoidea Taylor, Reference Taylor1910
Family Dictyocyathidae Taylor, Reference Taylor1910
Genus Graphoscyphia Debrenne in Zhuravleva, Reference Zhuravleva1974
?Graphoscyphia sp.
(Fig. 18g)
Material. Three specimens: MGM-7209X-1; MGM-7209X-12; MGM-7209X-14 (see Appendix 2 for localities).
Description. Cup 2.9–10 mm in diameter and intervallum 0.7–2.1 mm wide. Radial coefficient RK 7.8–9.1. Intervallar coefficient IK 0.15–0.34. Ratio of sides of interseptal loculi IC 1:3–1:4.3. Outer wall with 1–6 rows of pores per intersept (diameter 0.04 mm, lintels 0.02 mm, wall thickness 0.02–0.24 mm). Inner wall with one row of simple pores per intersept (diameter 0.16–0.20 mm, lintels 0. 08–0.28 mm, wall thickness 0.04–0.12 mm). Pseudosepta with 1–2? rows of pores (diameter 0.16 mm, lintels 0.08 mm, thickness 0.04–0.12 mm). Synapticulae are less regularly disposed.
Remarks. Our material presents the porosity of inner wall, septal porosity, synapticulae and septal arrangement similar to the specimen described by Debrenne Reference Debrenne1992 from EW, which in Kruse & Debrenne (Reference Kruse and Debrenne2020) is included in the synonymy as ?G. graphica.
Occurrence. Allochthonous clasts: Antarctica, Shackleton Range, Stephenson Bastion, Cenozoic glacial erratic tills. Cambrian Series 2, Botoman.
Superfamily Archaeocyathoidea Hinde, Reference Hinde1889
Family Archaeopharetridae Bedford & Bedford, Reference Bedford and Bedford1936
Genus Archaeopharetra Bedford & Bedford, Reference Bedford and Bedford1936
Archaeopharetra irregularis (Taylor, Reference Taylor1910)
(Figs 18e, h, 19a)
Reference Taylor1910 Dictyocyathus irregularis Taylor, p. 145, pl. 12, fig. 66.
Reference Debrenne, Zhuravlev, Kruse, Hooper and van Soest2002 Archaeopharetra irregularis (Taylor) – Debrenne, Zhuravlev & Kruse, p. 1665, fig. 62e, g–h.
Reference Debrenne, Zhuravlev and Kruse2012 Archaeopharetra irregularis (Taylor) – Debrenne, Zhuravlev & Kruse, p. 129, 132, fig. 103-1a-b.
Reference Debrenne, Zhuravlev, Kruse and Selden2015 Archaeopharetra irregularis (Taylor) – Debrenne, Zhuravlev & Kruse, p. 1051, 1054, fig. 626-1a-b.
Reference Perejón, Rodríguez-Martínez, Moreno-Eiris, Menéndez and Reitner2019 Archaeopharetra irregularis (Taylor) – Perejón et al., p. 17, fig. 9c, e, cum syn.
Material. Eleven specimens: MGM-7203X-4; MGM-7205X-1; MGM-7208X-2; MGM-7216X-11; MGM-7216X-12; MGM-7218X-27; MGM-7219X-9; MGM-7225X-3; MGM-7236X-4; MGM-7240X-6; MGM-7245X-1 (see Appendix 2 for localities).

Figure 19 (A) Archaeopharetra irregularis (Taylor, Reference Taylor1910): MGM-7245X-1, Stephenson Bastion. (B) Archaeocyathus sp.: MGM-7235X-16, Oldhamia Terraces. (C) ?Metacyathellus sp.: MGM-7208X-3, arrow points to subdivided pores, Stephenson Bastion. (D) Tabulaconus kordae Handfield, Reference Handfield1969: MGM-7223X-1, Swinnerton Ledge. (E–G) Archaeocyathina gen. et sp. indet.: (E) MGM-7204X-6, 7, 8, Stephenson Bastion; (F) MGM-7218X-42, Trueman Terraces; (G) MGM-7234X-5, Oldhamia Terraces. Scale bars = 2 mm (A–D, G); 1 mm (E, F).
Description. Conical cup, 0.8–5.5 mm in diameter. Intervallum 0.8–1.8 mm in width. Outer wall imperforate in the juvenile cups, and with 1–2 rows of centripetal pores in larger cups (wall thickness 0.04–0.16 mm). Inner wall with one row of simple pores per intersept (diameter 0,16–0.36 mm, lintels 0.20–0,28 mm, wall thickness 0,04–0.12 mm). Intervallum with coarsely porous pseudotaeniae linked by synapticulae, both skeletal elements are 0.04–0.08 mm in thickness.
Remarks. Shackleton specimens are similar to A. irregularis as described by Zhuravlev & Gravestock (Reference Zhuravlev and Gravestock1994) and Wrona & Zhuravlev (Reference Wrona and Zhuravlev1996).
Occurrence. Australia: Arrowie Basin, Ajax Mine, upper Ajax Limestone (Taylor Reference Taylor1910; Bedford & Bedford Reference Bedford and Bedford1936) and Moorowie Mine, Moorowie Formation (Lafuste et al. Reference Lafuste, Debrenne, Gandin and Gravestock1991). Stansbury Basin, Yorke Peninsula, Tepper's Knoll, Aquitaine SYC101 and Minlaton-1 drillholes, Koolywurtie Member of Parara Limestone, ‘Syringocnema favus beds’ (Zhuravlev & Gravestock Reference Zhuravlev and Gravestock1994). Allochthonous clasts: Africa, Namibia, Aranos Basin, Ganigobis, Botoman erratic cobbles in the Carboniferous Dwyka Group (Perejón et al. Reference Perejón, Rodríguez-Martínez, Moreno-Eiris, Menéndez and Reitner2019). Antarctica, Ellsworth Mts, Sentinel Range, Mt Lymburner, erratic clasts in the Permo-Carboniferous Whiteout Conglomerate Formation (Debrenne Reference Debrenne1992). King George Island, Admiralty Bay–Melville Peninsula, Oligocene Polonez Cove and Early Miocene Cape Melville Formation, glacio-marine deposits (Wrona & Zhuravlev Reference Wrona and Zhuravlev1996). Shackleton Range, Trueman Terraces and Oldhamia Terraces, carbonate clasts from the Mount Wegener Formation; Stephenson Bastion, Cenozoic glacial erratic tills. Cambrian Series 2, Botoman.
Family Archaeocyathidae Hinde, Reference Hinde1889
Genus Archaeocyathus Billings, Reference Billings1861
Archaeocyathus sp.
(Fig. 19b)
Material. One specimen: MGM-7235X-16 (see Appendix 2 for locality).
Description. Cup on oblique section of 4.1 × 11.2 mm in diameter. Outer wall with centripetal pores (diameter 0.16 mm; lintels 0.06 mm; 0.08–0.12 mm in thickness). Microporous sheath with 3–4 micropores over outer wall pore (diameter 0.04 mm; lintels 0.01 mm). Inner wall with one row of pores, bearing upwardly projecting, straight pores tubes. Intervallum with pseudotaenial network coarsely porous (0.16–0.24 mm in thickness).
Remarks. Different species of Archaeocyathus genus have been identified in a wide geographic range: Europe, Africa, Asia, America, Australia and Antarctica. Note among these the assignment to Archaeocyathus sp. has been made in material from South Africa, main Karoo Basin, Zwartskraal, Dwyka tillites (Debrenne Reference Debrenne1975; Debrenne & Kruse Reference Debrenne and Kruse1989) and in Antarctica, Ellsworth Mts, Heritage and Sentinel Ranges, erratic clasts in the Permo-Carboniferous Whiteout Conglomerate (Debrenne Reference Debrenne1992).
Occurrence. Allochthonous clasts: Antarctica, Shackleton Range, Oldhamia Terraces, carbonate clasts from the Mount Wegener Formation. Cambrian Series 2, Botoman.
Superfamily Metacyathoidea Bedford & Bedford, Reference Bedford and Bedford1934
Family Copleicyathidae Bedford & Bedford, Reference Bedford and Bedford1937
Genus Metacyathellus Debrenne & Zhuravlev, Reference Debrenne and Zhuravlev1990
?Metacyathellus sp.
(Fig. 19c)
Material. One specimen: MGM-7208X-3 (see Appendix 2 for locality).
Description. Cup on oblique section of 6.8 × 8.3 mm. Intervallum 2.5 mm in width. Outer wall with subdivided pores (diameter 0.08 mm, lintels 0.04 mm, wall thickness 0.04 mm). Inner wall with 1–2 rows of simple pores per intersept (diameter 0.16 mm, lintels 0.08 mm, wall thickness 0.16 mm). Intervallum with coarsely porous taeniae (0.08–0.12 mm in thickness). Vesicular tissue in intervallum and central cavity irregularly arranged.
Remarks. Our specimen presents a skeletal structure very similar to Metacyathellus caribouensis (Handfield) in Handfield (Reference Handfield1971, p. 64, pl. 11, fig. 2a) from Canada. Metacyathellus lairdi (Hill Reference Hill1964b) has been described in Antarctica, Nimrod Glacier (Hill Reference Hill1964b). The poor preservation of our material does not allow us to assign it with certainty to a specific species.
Occurrence. Allochthonous clasts: Antarctica, Shackleton Range, Stephenson Bastion, Cenozoic glacial erratic tills. Cambrian Series 2, Botoman.
Archaeocyathina gen. et sp. indet.
(Fig. 19e–g)
Material. Twelve specimens: MGM-7204X-4; MGM-7204X-6; MGM-7204X-7; MGM-7204X-8; MGM-7209X-20; MGM-7218X-42; MGM-7234X-5; MGM-7247X-1; MGM-7247X-2; MGM-7247X-3; MGM-7247X-5; MGM-7247X-11 (see Appendix 2 for localities).
Remarks. The small size of the cups and their state of preservation do not allow us to assign these samples to a specific genus and species.
Occurrence. Allochthonous clasts: Antarctica, Shackleton Range, Trueman Terraces, Oldhamia Terraces, carbonate clasts from the Mount Wegener Formation; Stephenson Bastion, Cenozoic glacial erratic tills. Cambrian Series 2, Botoman.
Phylum Cnidaria Verrill, Reference Verrill1865
Class Anthozoa Ehrenberg, Reference Ehrenberg1834
Subclass Zoantharia Scrutton, Reference Scrutton and House1979
Order Tabulaconida Scrutton, Reference Scrutton and House1979
Family Tabulaconidae Debrenne, Gangloff & Lafuste, Reference Debrenne, Gangloff and Lafuste1987
Genus Tabulaconus Handfield, Reference Handfield1969
Tabulaconus kordae Handfield, Reference Handfield1969
(Fig. 19d)
Reference Handfield1969 Tabulaconus kordae Handfield, p. 787, pl. 1, figs 2–5.
Reference Scrutton and House1979 Tabulaconus kordae Handfield – Scrutton, p. 179, fig. 2b (reproduced in Handfield Reference Handfield1969).
Reference Debrenne, Lafuste and Gangloff1981 Tabulaconus kordae Handfield – Debrenne, Lafuste & Gangloff, p.64.
Reference Rozanov, Hoffman and Nitecki1986 Tabulaconus kordae Handfield – Rozanov, fig. 28d.
Reference Debrenne, Gangloff and Lafuste1987 Tabulaconus kordae Handfield – Debrenne, Gangloff & Lafuste, p. 7–8, figs 5–10.
Reference Voronova, Drozdova, Esakova, Zhegallo, Zhuravlev, Rozanov, Sayutina and Ushatinskaya1987 Tabulaconus kordae Handfield – Voronova et al., p. 43, pl. 10, fig. 5.
Reference Zhuravlev1988 Tabulaconus kordae Handfield – Zhuravlev, p. 109, pl. 12, fig. 2.
Reference Mansy, Debrenne and Zhuravlev1993 Tabulaconus kordae Handfield – Mansy, Debrenne & Zhuravlev, pl. 1, fig. 1b, pl. 3, fig. 1.
Material. One specimen: MGM-7223X-1 (see Appendix 2 for locality).
Description. Solitary cup, 4 mm in height and 2.4 mm in diameter. Wall 0.01–0.04 mm thick with irregular undulations in the apical part. Inner cavity is crossed by thin tabulae (0.02 mm), most of which are complete, flat or arched upwards when they are recurved. Tabulae spacing ranges from 0.20 to 0.40 mm. Septa are not visible. The upper edge of cup is preserved.
Remarks. Our specimen shows the arrangement, distance and thickness of tabulae similar to the others described previously. Mansy et al. (Reference Mansy, Debrenne and Zhuravlev1993) figured and do not describe a small specimen like our cup. The preservation of the skeletal parts of our specimen is clearly different from that observed in the neighbouring archaeocyath cups (in the same thin section), being a differentiating feature of its primary skeletal composition. It is the first occurrence of this genus in Antarctica.
Occurrence. Canada: Northwest Territories, Sekwi Formation and British Columbia, Cassiar Mountains, Atan Group, co-occurrence with Polliaxis Zone (Handfield Reference Handfield1969); Mackenzie Mountains, Bonnia–Olenellus Zone (Voronova et al. Reference Voronova, Drozdova, Esakova, Zhegallo, Zhuravlev, Rozanov, Sayutina and Ushatinskaya1987); British Columbia, Rocky Mountains, Gataga River. Geological Survery of Canada locality 98754 (Mansy et al. Reference Mansy, Debrenne and Zhuravlev1993). USA, Alaska, Tatonduk River, Adams Argillite (Debrenne et al. Reference Debrenne, Gangloff and Lafuste1987). Russia, Koryakia, Koryak Highlands (Zhuravlev Reference Zhuravlev1988). Allochthonous clasts: Antarctica, Shackleton Range, Swinnerton Ledge, carbonate clasts from the Mount Wegener Formation. Cambrian Series 2, Botoman.
8. Biostratigraphical and palaeobiogeographical correlations
The stratigraphic distribution of the archaeocyathan genera from the Shackleton Range ranges from Tommotian 1 to Toyonian 3 (Siberian stages, Cambrian Stage 2 to Stage 4) according to Debrenne et al. (Reference Debrenne, Zhuravlev, Kruse and Selden2015) (see Appendix 3). There are no biozones based on archaeocyaths in Antarctica. The Antarctic archaeocyathan fauna has usually been compared with Australian species (see Debrenne & Kruse Reference Debrenne and Kruse1989; Wrona & Zhuravlev Reference Wrona and Zhuravlev1996) since many species are common and support the concept of a unified Australia–Antarctica province (Australian archaeocyath fauna from Arrowie and Stansbury basins, Gnalta Shelf and fauna from Antarctica, South Africa and Falkland Islands, according to Kruse & Shi in Brock et al. Reference Brock, Engelbretsen, Jago, Kruse, Laurie, Shergold, Shi, Sorauf, Wright, Young, Talent and Laurie2000). Thus, we compare the Shackleton Range species with the Australian biostratigraphic schemes. In Arrowie and Stansbury Basins, Australia, Zhuravlev & Gravestock (Reference Zhuravlev and Gravestock1994) designated three biozones (Warriootacyathus wilkawillinensis, Spirillicyathus tenuis and Jugalicyathus tardus) and informally named two younger biozones (‘Syringocnema favus beds’ and ‘Archaeocyathus abacus beds’).
Syringocnema favus beds were originally based on an assemblage that comprises 28 archaeocyathan species and two non-archaeocyathan taxa from Koolywurtie Member, Parara Limestone, Stansbury Basin (Zhuravlev & Gravestock Reference Zhuravlev and Gravestock1994). The Shackleton Range fauna can be closely correlated to the Syringocnema favus beds assemblage based on shared species: Tumuliolynthus irregularis, Nochoroicyathus hystrix, Nochoroicyathus lawrencei, Thalamocyathus trachealis, Erismacoscinus bilateralis, Coscinoptycta convoluta, Paranacyathus sarmaticus and Archaeopharetra irregularis. The Syringocnema favus beds were initially correlated with the Pararaia janeae Zone (Zhuravlev & Gravestock Reference Zhuravlev and Gravestock1994; Gravestock et al. Reference Gravestock, Alexander, Demidenko, Esakova, Holmer, Jago, Lin, Melnikova, Parkhaev, Rozanov, Yu, Ushatinskaya, Zang, Zhegallo and Zhuravlev2001; Jago et al. Reference Jago, Zang, Sun, Brock, Paterson and Skovsted2006), which belongs to the Australian trilobite zonation based on Jell in Bengtson et al. (Reference Bengtson, Conway Morris, Cooper, Jell and Runnegar1990). Other authors correlate the Syringocnema favus beds with the Pararaia bunyerooensis Zone (Paterson et al. Reference Paterson, Skovsted, Brock and Jago2007; Jago et al. Reference Jago, Gehling, Paterson, Brock and Zang2012, Reference Jago, Gehling, Betts, Brock, Dalgarno, García-Bellido, Haslett, Jacquet, Kruse, Langsford, Mount and Paterson2020; Kruse & Jago Reference Kruse and Jago2016; Betts et al. Reference Betts, Paterson, Jacquet, Andrew, Hall, Jago, Jagodzinski, Preiss, Crowley, Brougham, Mathewson, García-Bellido, Topper, Skovsted and Brock2018; Kruse & Debrenne Reference Kruse and Debrenne2020). Moreover, Kruse et al. (Reference Kruse, Zhuravlev, Parkhaev and Zhu2017) indicated that the archaeocyathan assemblage recognised in the Syringocnema favus beds is the most widespread in the Australia–Antarctica province, and some of their genera are restricted to the Botoman stage. Therefore, the Shackleton Range archaeocyath assemblage suggests a Botoman age ( = provisional upper Stage 3 and/or lower Stage 4) according to Peng et al. (Reference Peng, Babcock, Cooper, Gradstein, Ogg, Schmitz and Ogg2012), Ogg et al. (Reference Ogg, Ogg, Gradstein, Ogg, Ogg and Gradstein2016) and Geyer (Reference Geyer2019). The Botoman age is also confirmed by the presence of the excellently preserved coralomorph Tabulaconus kordae (Swinnerton Ledge, Mount Wegener Formation). This coralomorph is reported from Botoman fauna in the Koryak Highlands, Penzhina River basin, Russia, according to Zhuravlev (Reference Zhuravlev1988).
Kaltatocyathus gregarius and ?Baikalocyathus sp. are reported in the Atdabanian Spirillicyathus tenuis Zone in Australia (Gravestock Reference Gravestock1984), but they coexisted with Botoman taxa in the Shackleton Range (see Appendices 2 and 3). Thus, they expand their stratigraphic ranges in the Australia–Antarctica province.
Debrenne & Kruse (Reference Debrenne and Kruse1986) also reported the presence of Kymbecyathus avius from samples of central TAM and it was assigned questionably to the Atdabanian Jugalicyathus tardus Zone by Zhuravlev & Gravestock (Reference Zhuravlev and Gravestock1994), and may possibly be of Atdabanian age, rather than stated Botoman age, due to structural complexity (Kruse & Debrenne Reference Kruse and Debrenne2020, p. 53). In the Shackleton Range, K. avius occurs in a Cenozoic glacial erratic sample from Stephenson Bastion with other undetermined taxa. Therefore, it would have an uncertain ?Atdabanian–?Botoman stratigraphic range in the Shackleton Range (see Appendix 3).
In the Shackleton Range, a total of 189 specimens have been identified in the Mount Wegener Formation from Trueman Terraces (41), Swinnerton Ledge (8), Oldhamia Terraces (66) and in the Cenozoic erratics from the Stephenson Bastion (70) and Du Toit Nunataks (4) (see Appendix 2). The Shackleton Range archaeocyathan assemblage comprises 34 different taxa corresponding to five new genera, six new species, ten specific species, seven doubtful genera, 14 sp. and four gen. and sp. indeterminate. A new archaeocyath family has been proposed. Therefore, the Shackleton Range archaeocyathan fauna is one of the most diverse records of allochthonous Antarctic assemblages described so far.
The Mount Wegener Formation and the Cenozoic erratics share the following 9 species out of the total taxa described: Tumuliolynthus irregularis, Dokidocyathus sp., Nochoroicyathus lawrencei, Nochoroicyathus sp., Rotundocyathus glacius sp. nov., Ladaecyathus sp., Shackletoncyathus buggischi gen. et sp. nov., Santelmocyathus santelmoi gen. et sp. nov. and Archaeopharetra irregularis. The archaeocyaths that only occur in the Mount Wegener Formation are Kaltatocyathus gregarius, Cadniacyathus sp., Buggischicyathus microporus gen. et sp. nov., ?Baikalocyathus sp., ?Fallocyathus sp., ?Antoniocoscinus sp., Erismacoscinus bilateralis, ?Retecoscinus sp., Wegenercyathus sexangulae gen. et sp. nov., Coscinoptycta convoluta, Putapacyathus sp., Paranacyathus sarmaticus and Archaeocyathus sp. The taxa that are only recorded in Cenozoic erratics from the Stephenson Bastion and Du Toit Nunataks are Kymbecyathus avius, Nochoroicyathus hystrix, Thalamocyathus trachealis, Paragnaltacyathus hoeflei gen. et sp. nov., ?Ussuricyathellus sp., Neoloculicyathus sp., ?Graphoscyphia sp. and ?Metacyathellus sp. (see Appendix 3).
This study describes the first reported occurrence of Kaltatocyathus, Rotundocyathus, ?Baikalocyathus, ?Ussuricyathellus, ?Fallocyathus, ?Antoniocoscinus, ?Retecoscinus and Neoloculicyathus in Antarctica. It also undoubtedly confirms the presence of Cadniacyathus. Tumulocyathus (?Tumulocyathus) curvatus sp. nov. was previously described by Hill (Reference Hill1965) from the Whichaway Nunataks, and has subsequently been reassigned to ?Cadniacyathus curvatus by Debrenne & Kruse (Reference Debrenne and Kruse1989).
8.1. Comparison with autochthonous archaeocyathan assemblages
These new records in Antarctica allow the establishment of new paleobiogeographic relationships (Fig. 20). In Australia, the occurrence of Cadniacyathus and Rotundocyathus had only been reported in the Ajax Limestone, Ajax Mine, Arrowie Basin (Bedford & Bedford Reference Bedford and Bedford1937; Debrenne Reference Debrenne1974b; Kruse & Debrenne Reference Kruse and Debrenne2020). Ussuricyathellus has been described in the White Point Conglomerate, Stansbury Basin, Kangaroo Island (Kruse & Moreno-Eiris Reference Kruse and Moreno-Eiris2013) and Ajax Mine, Arrowie Basin (Kruse & Debrenne Reference Kruse and Debrenne2020), Australia, as well as in the Burgasutay Formation, Seer Mountains, western Mongolia (Voronin Reference Voronin1988). Fallocyathus has been described in Pestrotsvet Formation, Siberian Platform, Russia (Zhuravleva et al. Reference Zhuravleva, Korshunov, Rozanov and Zhuravleva1969) and from the Santo Domingo Formation, Córdoba, Iberia (Perejón et al. Reference Perejón, Moreno-Eiris and Menéndez2008).

Figure 20 (A) Schematic geologic map of the Antarctic outcrops (modified from Tingey Reference Tingey1991). (B) Distribution of the archaeocyathan assemblages that have been previously reported and/or studied. Key: 1 = Debrenne & Kruse (Reference Debrenne and Kruse1986); 2 = Hill (Reference Hill1964b); Debrenne & Kruse (Reference Debrenne and Kruse1986, Reference Debrenne and Kruse1989)(; 3 = Hill (Reference Hill and Adie1964a); 4 = Konyushkov & Shulyatin (Reference Konyushkov, Shulyatin and Zhuravleva1980); 5 = Wood et al. (Reference Wood, Evans and Zhuravlev1992); 6 = Hill (Reference Hill1965); 7 = Höfle & Buggisch (Reference Höfle and Buggisch1995); Buggisch & Henjes-Kunst (Reference Buggisch and Henjes-Kunst1999); 8 = Debrenne et al. (Reference Debrenne, Rozanov and Webers1984); Henderson et al. (Reference Henderson, Debrenne, Rowell, Webers, Webers, Craddock and Splettstoesser1992); 9 = Debrenne (Reference Debrenne1992); 10 = Morycowa et al. (Reference Morycowa, Rubinowski and Tokarski1982); Wrona (Reference Wrona1989); Wrona & Zhuravlev (Reference Wrona and Zhuravlev1996); 11 = Gordon (Reference Gordon1920). Abbreviations: AP = Antarctic Peninsula; EWB = Ellsworth–Whitmore Block; MBL = Marie Byrd Land; TAM = Transantarctic Mountains; DML = Dronning Maud Land; SR = Shackleton Range; Gl. = glacier.
The remaining newly reported genera from the Shackleton Range (?Baikalocyathus, ?Antoniocoscinus, ?Retecoscinus and Neoloculicyathus) have a wide geographical distribution. However, the Shackleton Range fauna have three species that have only been described in Australia – Kaltatocyathus gregarius (Arrowie Basin; Gravestock Reference Gravestock1984), Nochoroicyathus hystrix and Nochoroicyathus lawrencei (Gnalta Shelf; Kruse Reference Kruse1982) (see Appendix 4).
The Shackleton Range fauna show an extremely limited specific affinity with the autochthonous fauna from Antarctica. A total of 45 species have been previously described in the early Cambrian record of Antarctica, including 37 from the Shackleton Limestone, central TAM (Hill Reference Hill1964b; Debrenne & Kruse Reference Debrenne and Kruse1986, Reference Debrenne and Kruse1989; 1 and 2 in Fig. 20) and 17 from the Schneider Hills limestone, Argentina Range (Konyushkov & Shulyatin Reference Konyushkov, Shulyatin and Zhuravleva1980; Debrenne & Kruse Reference Debrenne and Kruse1989; 4 in Fig. 20). However, only Kymbecyathus avius (Shackleton Limestone) and Thalamocyathus trachealis (Shackleton Limestone and Schneider Hills limestone) are in common with the Shackleton Range assemblage (see Appendix 4).
The Shackleton Range archaeocyathan assemblage shares nine species and 21 genera with the Australian fauna. It shares eight species with the fauna of Arrowie Basin: Tumuliolynthus irregularis, Kaltatocyathus gregarius, Nochoroicyathus cf. lawrencei, Thalamocyathus trachealis, Erismacoscinus bilateralis, Coscinoptycta convoluta, Paranacyathus sarmaticus and Archaeopharetra irregularis (Etheridge Reference Etheridge1890; Taylor Reference Taylor1910; Bedford & Bedford Reference Bedford and Bedford1934, Reference Bedford and Bedford1936; Debrenne & Debrenne Reference Debrenne and Debrenne1960; Debrenne Reference Debrenne1969, Reference Debrenne1974a, Reference Debrenne1974b; Daily Reference Daily1973; Lafuste et al. Reference Lafuste, Debrenne, Gandin and Gravestock1991; Zhuravlev & Gravestock Reference Zhuravlev and Gravestock1994; Kruse & Debrenne Reference Kruse and Debrenne2020); one with Amadeus and Georgina Basins: Erismacoscinus bilateralis (Kruse & West 1980); five with Gnalta Shelf: Tumuliolynthus irregularis, Nochoroicyathus hystrix, Nochoroicyathus lawrencei, Coscinoptycta convoluta and Archaeopharetra irregularis (Kruse Reference Kruse1978, Reference Kruse1982); and six with Stansbury Basin: Tumuliolynthus irregularis, Kaltatocyathus aff. gregarius, Thalamocyathus trachealis Erismacoscinus bilateralis, Coscinoptycta convoluta and Archaeopharetra irregularis (Gravestock Reference Gravestock1984; Debrenne & Gravestock Reference Debrenne and Gravestock1991; Zhuravlev & Gravestock Reference Zhuravlev and Gravestock1994; Kruse & Moreno-Eiris Reference Kruse and Moreno-Eiris2013) (see Appendix 4).
8.2. Comparison with allochthonous archaeocyathan assemblages
The Shackleton Range fauna only share ?Ladaecyathus and ?Erismacoscinus with the allochthonous archaeocyathan record from the marine Cambrian El Jagüelito Formation in South America (González et al. Reference González, Tortello and Damborenea2011 and references therein).
The Shackleton Range archaeocyathan assemblage shows greater affinity with the allochthonous assemblages described in Permo-Carboniferous tillites of the Australia–Antarctica province. The Shackleton Range fauna share five of the eight species described in the Permo-Carboniferous Whiteout Conglomerate, Ellsworth Mountain, Antarctica (Debrenne Reference Debrenne1992; 9 in Fig. 20): Erismacoscinus cf. bilateralis, Paranacyathus, ?Graphoscyphia graphica, Archaeopharetra irregularis and Archaeocyathus sp. The number of described genera from the Falkland Islands (Stone et al. Reference Stone, Thomson and Rushton2012), the main Karoo Basin (Debrenne Reference Debrenne1975), the Aranos Basin (Perejón et al. Reference Perejón, Rodríguez-Martínez, Moreno-Eiris, Menéndez and Reitner2019) and the Sierras Australes (González et al. Reference González, Tortello, Damborenea, Naipauer, Sato and Varela2013) is 16, 13, 12 and 1, respectively. The Falkland Islands (Stone et al. Reference Stone, Thomson and Rushton2012), main Karoo Basin (Debrenne Reference Debrenne1975), Aranos Basin (Perejón et al. Reference Perejón, Rodríguez-Martínez, Moreno-Eiris, Menéndez and Reitner2019) and the Sierras Australes (González et al. Reference González, Tortello, Damborenea, Naipauer, Sato and Varela2013) share three (Tumuliolynthus, Thalamocyathus and ?Erismacoscinus), four (Thalamocyathus, ?Ladaecyathus, Archaeopharetra and Archaeocyathus), three (Erismacoscinus, Graphoscyphia and Archaeopharetra) and one (?Thalamocyathus) genera with the Shackleton Range, respectively (see Fig. 1 and Appendix 4).
The archaeocyath-bearing clasts in Cenozoic glacial erratics are very common in Antarctica. It should be noted that the Shackleton Range fauna have only one common species with the Beardmore Glacier assemblage reported from present moraines in central TAM (Hill Reference Hill and Adie1964a; 3 in Fig. 20) – Coscinoptycta convoluta. However, the highest affinity is with the Cenozoic deposits of King George Island, the Oligocene Polonez Cove (Morycowa et al. Reference Morycowa, Rubinowski and Tokarski1982; Wrona & Zhuravlev Reference Wrona and Zhuravlev1996) and the Miocene Cape Melville Formations (Wrona & Zhuravlev Reference Wrona and Zhuravlev1996) (10 in Fig. 20), sharing Tumuliolynthus irregularis, Thalamocyathus trachealis, Erismacoscinus, Putapacyathus, Paranacyathus sarmaticus, Archaeopharetra irregularis and ?Archaeocyathus from a total of 28 species described. ?Nochoroicyathus, ?Cadniacyathus, Thalamocyathus trachealis, Erismacoscinus bilateralis, Putapacyathus, ?Graphoscyphia and Archaeocyathus have been also reported from present-day moraines in the Whichaway Nunataks, the closest allochthonous record to the Shackleton Range, where an assemblage of 24 species has been described (Hill Reference Hill1965; 6 in Fig. 20) (see Appendix 4).
In Cenozoic Weddel Sea gravels (11 in Fig. 20), Gordon (Reference Gordon1920) described an archaeocyath assemblage of 14 taxa where Thalamocyathus trachealis?, Erismacoscinus bilateralis, ?Graphoscyphia and Archaeopharetra are in common with the Shackleton Range fauna.
9. Cambrian synorogenic record of the Shackleton Range and new age constraints on the Mount Wegener Formation
The Cambrian record currently crops out at the Shackleton Range as part of the Mount Wegener Formation, as Cenozoic glacial erratics derived from the Mount Wegener Formation (Stephenson Bastion and Du Toit Nunataks), and as locally sourced middle Cambrian erratics (trilobite shales) in the Mount Provender area (MP in Fig. 4a). Thus, all the information on the ages and sedimentary data of the Cambrian synorogenic marine sedimentation in the Shackleton Range sector (a-b in Fig. 21) comes from these units. Subsequently, the tectonic evolution of the Shackleton Range sector is synthesised, and possible synorogenic Cambrian sedimentation ages are reviewed and discussed.

Figure 21 Cambrian paleogeography before the final amalgamation of Gondwana (modified from Boger & Miller Reference Boger and Miller2004) showing the main cratonic areas, the location of the Shackleton Range sector (a-b), and the localities with Cambrian carbonate platform inboard sequences in the Pacific (initially passive) margin of Antarctica (PM, TAM) and Australia. The hypothetical seaways of the associated Ediacaran Mozambique and Cambrian Kuunga sutures are depicted. The so-called ‘Northern Terrane’ (see the text) is represented, but not the outboard terranes of Antarctica, Australia or South America. (a-b, c-d) Comparison of the geodynamic settings during synorogenic Cambrian sedimentation in the Shackleton Range sector (a-b, after Buggisch et al. Reference Buggisch, Kleinschmidt, Kreuzer and Krumm1990; Kleinschmidt & Buggisch Reference Kleinschmidt and Buggisch1994) and in the central Transantarctic Mountains sector (c-d, modified from Goodge Reference Goodge2020). In the Shackleton Range, the clasts from the Mount Wegener Formation and those from this unit present in the Cenozoic glacial tills suggest the existence of a volcanic arc and mixed sediment inputs of Ediacaran and Cambrian age (Terreneuvian Series 2). However, Terreneuvian Series 2 shallow-water deposits on the Proterozoic Watts Needle Formation or their deep-water equivalents are unknown. Low sedimentation rates or even erosion at the EAC margin during that time cannot be ruled out. In this study we propose the reconstruction of a lost Cambrian carbonate platform (see Fig. 22) in the Shackleton Range sector. In the central Transantarctic Mountains sector (c-d), the Series 2 Shackleton Limestone represents the platform development during the passive margin stage, while the Starshot Formation and the Douglas Conglomerate reflect syn- to late orogenic sedimentation (see Goodge Reference Goodge2020). Abbreviations: SA = South America; A = Africa; K = Kalahari craton; D = Dharwar craton; AU = Australia; P = Pilbara craton; Y = Yilgarn craton; Ga = Gawler craton; G = Grunehogne craton; EAC = East Antarctic craton; TA = Terre Adélie craton = Antarctic equivalent of Gawler craton that forms the ‘Mawson continent’ extending towards the Miller Range and Read Mountains; SPC = South Prince Charles Mountains; SR = Shackleton Range; PM = Pensacola Mountains; TAM = Transantarctic Mountains. The current contours of SR, PM and TAM are shown with a dashed grey line.

Figure 22 Hypothetical reconstruction of a hidden carbonate platform that was one of the source areas of upper slope to basinal facies of the Mount Wegener Formation. The combined analysis of microfacies, diagenesis and archaeocyaths from the carbonate clasts (Mount Wegener Formation and Cenozoic tills) allows differentiation between well-defined sedimentary sub-environments (1–9) in a lost Cambrian mixed siliciclastic–carbonate platform developed in a volcanic arc during the final amalgamation of E and W Gondwana. Abbreviations: Aj = Ajacicyathidae; De = Densocyathidae; Br = Bronchocyathidae; Ka = Kaltatocyathidae; Ky = Kymbecyathidae; Di = Dictyocyathidae; Co = Copleicyathidae; CBA = Calcimicrobial boundstones with archaeocyaths; CAB = Calcimicrobe–archaeocyath boundstones; AC = Archaeocyath cementstones; Ep = Epiphyton; An = Angusticellularia; Pr = Proaulopora; Re = Renalcis; MWF = Mount Wegener Formation; Lmst = limestones; Dol = dolostones; St = sandstones; IR = igneous rocks; MR = metamorphic rocks; VR = acidic to basic volcanic rocks; Ty 1–Ty 3 = ooid types; Agg = aggregate grains; Soci = sandy oolitic compound intraclasts; Int = intraclasts; Arch = archaeocyaths in muddy bottoms; Tri = trilobites; Bra = brachiopods; Ech = echinoderms; Cor = coralomorphs; Ch = chancelloriids; Hyo = hyoliths; Spm = sponge megascleres.
The Mount Wegener Formation consists of marine clastic deposits sedimented from the upper slope to deep basinal settings in synorogenic conditions (Buggisch & Henjes-Kunst Reference Buggisch and Henjes-Kunst1999). The high proportion of plagioclase, volcanoclastic and unstable heavy minerals suggest that the lower Cambrian Mount Wegener Formation was deposited in a back-arc basin (Buggisch et al. Reference Buggisch, Kleinschmidt, Kreuzer and Krumm1990; Kleinschmidt & Buggisch Reference Kleinschmidt and Buggisch1994; Buggisch et al. Reference Buggisch, Kleinschmidt, Höhndorf and Pohl1994a). However, the carbonate platform that generated the clasts that eventually formed the conglomerates and breccias of this formation does not outcrop in the Shackleton Range. Furthermore, this concealed platform developed on the Northern Belt, northwards of the Read Group (part of the EAC) and its sedimentary cover (Watts Needle Formation) (Fig. 3a; b in Fig. 21).
The Shackleton Range is interpreted as a composite terrane by Will et al. (Reference Will, Zeh, Gerdes, Frimmel, Millar and Schmädicke2009, Reference Will, Frimmel, Zeh, Le Roux and Schmädicke2010) based on uranium–lead (U–Pb) zircon and monazite ages, geochemical and isotope data. The southern terrane would show similar characteristics to the Mawson Continent (Will et al. Reference Will, Zeh, Gerdes, Frimmel, Millar and Schmädicke2009; Boger Reference Boger2011). In the Northern Terrane (Fig. 21), the magmatism associated with the subduction of the oceanic crust occurred at ~530 Ma (Zeh et al. Reference Zeh, Millar, Kroner and Görz1999; Will et al. Reference Will, Zeh, Gerdes, Frimmel, Millar and Schmädicke2009). The K–Ar amphibole cooling ages from the ophiolitic complex, a relic of the Mozambique Ocean? (Tessensohn et al. Reference Tessensohn, Kleinschmidt, Talarico, Buggisch, Brommer, Henjes-Kunst, Kroner, Millar and Zeh1999a), oscillate between 510 and 490 Ma (Talarico et al. Reference Talarico, Kleinschmidt and Henjes-Kunst1999). In addition, the final continent–continent collision with eclogite facies metamorphism of 800–850 °C/23–25 kbar at approximately 70 km depth (Schmädicke & Will Reference Schmädicke and Will2006; Romer et al. Reference Romer, Mezger and Schmädicke2009) developed at 525–520 Ma (ultramafic rocks, Romer et al. Reference Romer, Mezger and Schmädicke2009) and ~510 Ma (felsic country rocks, Will et al. Reference Will, Zeh, Gerdes, Frimmel, Millar and Schmädicke2009). Thus, ultramafic–mafic rocks and ophiolitic relics are related to the final amalgamation between E and W Gondwana (Tessensohn et al. Reference Tessensohn, Kleinschmidt, Talarico, Buggisch, Brommer, Henjes-Kunst, Kroner, Millar and Zeh1999a; Kleinschmidt et al. Reference Kleinschmidt, Henjes-Kunst and Tessensohn2001; Schmädicke & Will Reference Schmädicke and Will2006; Will et al. Reference Will, Zeh, Gerdes, Frimmel, Millar and Schmädicke2009, Reference Will, Frimmel, Zeh, Le Roux and Schmädicke2010). In fact, different hypothetical traces of the E–W Gondwana sutures have been suggested (Moyes et al. Reference Moyes, Groenewald and Brown1993; Grunow et al. Reference Grunow, Hanson and Wilson1996; Shackleton Reference Shackleton1996; Jacobs et al. Reference Jacobs, Fanning, Henjes-Kunst, Olesch and Paech1998, Reference Jacobs, Opås, Elburg, Läufer, Estrada, Ksienzyk, Damaske and Hofmann2017; Fitzsimons Reference Fitzsimons2000; Yoshida et al. Reference Yoshida, Jacobs, Santosh and Rajesh2003; Boger & Miller Reference Boger and Miller2004; Schmädicke & Will Reference Schmädicke and Will2006; Kleinschmidt & Boger Reference Kleinschmidt and Boger2009, among others).
In the Northern Belt of the Shackleton Range, the Precambrian basement rocks with Pan-African overprinting and the northern neighbouring Coats Land Block (Kleinschmidt & Boger Reference Kleinschmidt and Boger2009; Loewy et al. Reference Loewy, Dalziel, Pisarevsky, Connelly, Tait, Hanson and Bullen2011) are separated from the EAC (northernmost part of the Mawson Continent, Will et al. Reference Will, Zeh, Gerdes, Frimmel, Millar and Schmädicke2009; Boger Reference Boger2011) by an E–W suture. Aeromagnetic data suggest that the E–W Shackleton Range suture could extend at least 500 km into E Antarctica and shift to an N–S orientation in the Recovery Lakes area (Golynsky et al. Reference Golynsky, Ferraccioli, Hong, Golynsky, von Frese, Young, Blankenship, Holt, Ivanov, Kiselev, Masolov, Eagles, Gohl, Jokat, Damaske, Finn, Aitken, Bell, Armadillo, Jordan, Greenbaum, Bozzo, Caneva, Forsberg, Ghidella, Galindo-Zaldivar, Bohoyo, Martos, Nogi, Quartini, Kim and Roberts2018). It should be noted that the Coats Land Block is separated from the Kalahari and Grunehogne cratons (K and G in Fig. 21) by the Grenvillian-age Maud Belt, which is interpreted as the continuation of the Namaqua-Natal Belt of southernmost Africa (Jacobs et al. Reference Jacobs, Fanning and Bauer2003; Wang et al. Reference Wang, Jacobs, Elburg, Läufer, Thomas and Elvevold2020, fig. 11).
The sedimentation age of the Mount Wegener Formation has been estimated in different ways (Fig. 3). Shales give an Rb–Sr isochron age of ~526 Ma that was interpreted as a pre-cleavage event, such as diagenesis of sediments (Pankhurst et al. Reference Pankhurst, Marsh, Clarkson, Oliver, James and Jago1983). K–Ar dating ages around 547–506 Ma (2–6 μm whole rock fraction) were interpreted as mixtures of inherent sedimentation/diagenesis ages and around 490 Ma as the upper limit of deformation and metamorphism (Buggisch et al. Reference Buggisch, Kleinschmidt, Kreuzer and Krumm1994b). However, the K–Ar dating of detrital muscovites from greywacke turbidites of the Mount Wegener Formation ranges between 572 and 534 Ma, reflecting source rocks with different Pan-African primary cooling and exhumation histories; thus, the minimum cooling age limited a maximum age of sedimentation of the Mount Wegener Formation up to ca. 535 Ma (Buggisch & Henjes-Kunst Reference Buggisch and Henjes-Kunst1999). In fact, this minimum cooling age of detrital muscovites matches with the age of magmatism in the northern terrane, which is associated with subduction of oceanic crust by ~530 Ma (Zeh et al. Reference Zeh, Millar, Kroner and Görz1999; Will et al. Reference Will, Zeh, Gerdes, Frimmel, Millar and Schmädicke2009). Therefore, the Rb–Sr and K–Ar dating ages support an unknown Ediacaran–Terreneuvian rock source that does not crop out in the Shackleton Range (Figs 3, 21). The only known Ediacaran rocks are the EAC's autochthonous sedimentary cover, Watts Needle Formation. However, the paleocurrents of the Mount Wegener Formation point to a source area located to the N (Buggisch & Henjes-Kunst Reference Buggisch and Henjes-Kunst1999), whereas the paleocurrents from the Watts Needle Formation point to the S (Buggisch et al. Reference Buggisch, Kleinschmidt, Kreuzer and Krumm1990). Furthermore, the neodymium (Nd) isotope values of the Mount Wegener Formation are more like Pan-African Grenville-age basement rocks such as Pioneers Group, than those from the Read Group (Buggisch & Henjes-Kunst Reference Buggisch and Henjes-Kunst1999).
The fossil content of the Mount Wegener Formation was analysed in an incipient way (Buggisch et al. Reference Buggisch, Kleinschmidt, Kreuzer and Krumm1990, Reference Buggisch, Kleinschmidt, Höhndorf and Pohl1994a), so the previous given age is a wide early Cambrian Atdabanian age (Buggisch & Henjes-Kunst Reference Buggisch and Henjes-Kunst1999). It should also be considered that there are two groups of fossils, one within the allochthonous carbonate clasts in the conglomerates, and another in the autochthonous presence of the Oldhamia ichnotaxon on the turbidite levels (Oldhamia cf. antiqua and Oldhamia cf. radiata according to Buggisch et al. Reference Buggisch, Kleinschmidt, Kreuzer and Krumm1990). The First Appearence Datum (FAD) of Oldhamia is placed in the Fortunian (Mángano & Buatois Reference Mángano, Buatois, Mángano and Buatois2016). However, Herbosch & Verniers (Reference Herbosch and Verniers2011) reviewed the biostratigraphic value of the cosmopolitan Cambrian Oldhamia ichnospecies and suggested that of 19 occurrences observed worldwide, 14 (Mount Wegener Formation included) occurred in a well-constrained time interval, ranging from the base of Stage 3 to the lower three quarters of Wuliuan. Furthermore, these authors conclude that Oldhamia taxa from the Mount Wegener Formation just above the archaeocyaths, have an age that could extend from the base of Stage 3 (appearance of trilobites) to the lower half of Stage 4 (high diversity of archaeocyaths). The stratigraphic section of the Mount Wegener Formation records around 770 m at the Trueman Terraces but probably exceeds 1000 m (see Figs 4d, 5), although the total thickness of the unit is unknown. Oldhamia is recorded in the intermediate levels of the Oldhamia Terraces. However, we cannot rule out the presence of archaeocyath-bearing clasts or ichnotaxa in the rest of the mapped thickness of the Mount Wegener Formation (see Fig. 4d) since polymictic conglomerates crop out throughout the Oldhamia Terraces and because the exact position of the studied samples from Oldhamia Terraces is unknown.
Furthermore, from analysis of detrital zircons, MacNaughton et al. (Reference MacNaughton, Moynihan, Roots and & Crowley2016) determined a younger, early Guzhangian age limit for new occurrences of Cambrian Oldhamia ichnospecies (O. antiqua, O. curvata and O. flabellata) in the Selwyn Basin, Canada. They also suggested a possible Last Appearance Datum (LAD) for O. radiata in the top of the Arrowhead Lake Member. This LAD for O. radiata would be below the basal conglomerate of the Gull Lake Formation, in the transition between the Nevadella and Bonnia–Olenellus Zones. The basal limestone conglomerate contains archaeocyath- and Tabulaconus-bearing clasts that may represent debris flows from coeval platform deposits of the Sekwi Formation (Gordey & Anderson Reference Gordey and Anderson1993). In fact, this basal limestone conglomerate is correlated by MacNaughton et al. (Reference MacNaughton, Moynihan, Roots and & Crowley2016) with the regressive sandstone found within the Sekwi Formation in the Mackenzie Mountains, Northwest Territories. The Sekwi Formation contains trilobites from the Fallotaspis, Nevadella and Bonnia–Olenellus Zones (Fritz Reference Fritz1972) and three Botoman archaeocyath assemblages (archaeocyathan zonation for Laurentia according to Mansy et al. Reference Mansy, Debrenne and Zhuravlev1993, modified by McMenamin et al. Reference McMenamin, Debrenne and Zhuravlev2000). Lower Botoman Ethmophyllum withneyi–Sekwicyathus nahanniensis belonging to the middle Nevadella strata and two middle Botoman assemblages Claruscoscinus fritzi–M. caribouensis and ?Pycnoidocoscinus serratus–Tabulaconus kordeae occurring within the middle Bonnia–Olenellus strata. Specifically, the carbon isotopic excursion cycle C recorded in the uppermost Nevadella Zone in the Sekwi Formation has been correlated with the VII positive excursion on the Siberian carbon isotope curve by Dilliard et al. (Reference Dilliard, Pope, Coniglio, Hasiotis and Lieberman2007). Harvey et al. (Reference Harvey, Williams, Condon, Wilby, Siveter, Rushton, Leng and Gabbott2011) provided a maximum age of 514.45 ± 0.36 Ma for the Cambrian Stage 3–Stage 4 boundary with the zircon 206Pb/238U dating of an ash from the upper part of the trilobite Callavia biozone (England). On the Siberian Platform, He et al. (Reference He, Zhu, Mills, Wyn, Zhuravlev, Tostevin, Pogge von Strandmann, Yang, Poulton and Shields2019) correlated the boundary of the uppermost Atdabanian archaeocyath Fansycyathus lermontovae Zone and the lowermost Botoman trilobite Bergeroniellus micmaciformis–Erbiella Zone with this radiometric age. Thus, the age of the Atdabanian/Botoman boundary remains uncertain on the Siberian Platform. The VII positive C-isotope excursion is recorded in the basal Botoman trilobile B. micmaciformis–Erbiella Zone, around 514 Ma (according to He et al. Reference He, Zhu, Mills, Wyn, Zhuravlev, Tostevin, Pogge von Strandmann, Yang, Poulton and Shields2019). Thus, in the Selwyn Basin, O. radiata was coeval with lower Botoman archaeocyaths but did not coexist with middle Botoman archaeocyath assemblages or with the coralomorph Tabulaconus kordeae.
It is noteworthy to mention that the Cambrian fauna of the Mount Wegener Formation shares three species with the fauna of the Selwyn Basin: O. radiata, O. antiqua and Tabulaconus kordeae. The archaeocyathan fauna from the limestone clasts of the Mount Wegener Formation provide a correlation with Botoman fauna (see section 8) for the carbonate platform from which they were derived. Specifically, the archaeocyath assemblage of the Shackleton Range shares taxa with the Syringocnema favus beds fauna (see section 8). This archaeocyathan fauna is time-equivalent to the trilobite Pararaia bunyerooensis Zone (see Jago et al. Reference Jago, Gehling, Betts, Brock, Dalgarno, García-Bellido, Haslett, Jacquet, Kruse, Langsford, Mount and Paterson2020 and references therein), and the radiometric ("chemical abrasion" or CA-TIMS method ) ages of three volcanic horizons within the P. bunyerooensis Zone from the Mernmerna Formation support ages of 514.46 ± 0.13 Ma, 514.56 ± 0.13 Ma and 515.38 ± 0.13 Ma (Betts et al. Reference Betts, Paterson, Jacquet, Andrew, Hall, Jago, Jagodzinski, Preiss, Crowley, Brougham, Mathewson, García-Bellido, Topper, Skovsted and Brock2018). The archaeocyath-bearing clasts from the Mount Wegener Formation were deposited on upper slope to basinal settings after the breaking up and sedimentary brecciation of the carbonate platform, just after early marine phreatic to vadose diagenesis (see section 6). Therefore, at least the first 650–700 m of thickness (Fig. 5a) can be assigned to a maximum depositional age of terminal Stage 3 (~515.5–514.3 Ma according to Australian data from Betts et al. Reference Betts, Paterson, Jacquet, Andrew, Hall, Jago, Jagodzinski, Preiss, Crowley, Brougham, Mathewson, García-Bellido, Topper, Skovsted and Brock2018). The Shackleton Range record shows that O. radiata was partially contemporaneous with Tabulaconus kordeae and suggests certain diachronism with the O. radiata LAD from the Selwyn Basin. There are insufficient data to establish the final sedimentation age of the Mount Wegener Formation beyond the Wuliuan (according Herbosch & Verniers Reference Herbosch and Verniers2011) or beyond the early Guzhangian considering the LAD of Cambrian Oldhamia ichnospecies proposed by MacNaughton et al. (Reference MacNaughton, Moynihan, Roots and & Crowley2016). Therefore, the minimum age of the unit is given by the deformation and metamorphism ages around 490 Ma (Buggisch et al. Reference Buggisch, Kleinschmidt, Kreuzer and Krumm1994b) during Furongian.
In the Shackleton Range, the middle Cambrian erratics are fossiliferous shales and calcareous siltstones containing trilobites, obolid brachiopods, hyoliths and other molluscs, and are informally known as the ‘trilobite shales’ (Fig. 3; see Thomson et al. Reference Thomson, Solov'ev, Buggisch, Thomson and Thomson1995 and references therein). Solov'ev & Grikurov (Reference Solov'ev and Grikurov1979) described nine trilobite assemblages from these erratics. The presence of the brachiopod Notiobolus tenuis (Popov & Solov'ev Reference Popov and Solov'ev1981) was assigned to a pre-Drumian age based on its co-occurrence with Ptychagnostus gibbus and Ptychagnostus praecurrens (Popov et al. Reference Popov, Holmer, Hughes, Ghobadi Pour and Myrow2015). Solov'ev and Grikurov's trilobite associations have been grouped as Fauna 2 and correlated with gibbus to atavus Zones, from late Templetonian to early Floran Australian stages by Cooper & Shergold (Reference Cooper, Shergold and Tingey1991). However, Lieberman (Reference Lieberman2004) suggested that some of the Solov'ev & Grikurov (Reference Solov'ev and Grikurov1979) figured material might be referred only questionably to Ammagnostus laiwuensis, showing a broad range in South China from the upper Ptychagnostus atavus Zone to the Proagnostus bulbus Zone (Peng & Robison Reference Peng and Robison2000), late Floran to early Mindyallan (Drumian–Guzhangian). Therefore, the trilobite shales were deposited during the Wuliuan–Drumian or even Wuliuan–?Guzhangian, depending on whether Lieberman's subsequent reassignment is correct. Trilobite distortion and K–Ar ages (2–6 μm) of shales around 463–455 Ma are interpreted as weak deformation and very low-grade metamorphism (Buggisch et al. Reference Buggisch, Kleinschmidt, Höhndorf and Pohl1994a). Thus, the stratigraphic position of this ex situ record has been placed under the sedimentary molasse deposits of the Ordovician Blaiklock Glacier Group (Fig. 3; Thomson et al. Reference Thomson, Solov'ev, Buggisch, Thomson and Thomson1995). The Blaiklock Glacier Group has yielded an Rb–Sr date of 482 ± 11 Ma isochron (Pankhurst et al. Reference Pankhurst, Marsh, Clarkson, Oliver, James and Jago1983), while the K–Ar date of micas from underlying leucogneiss, undeformed granitic clast and detrital micas from sandstones are around 516–498 Ma with palaeomagnetic declination data according to known Ordovician pole positions (Buggisch et al. Reference Buggisch, Bachtadse and Henjes-Kunst1999). Thus, the detrital micas from the Blaiklock Glacier Group support a Guzhangian–Furongian uplift and a history of exhumation for the northern basement of the Shackleton Range.
In summary, part of the upper slope to deep basinal deposits from the Mount Wegener Formation contains evidence of rock sources from late Ediacaran up to Cambrian Series 2, and locally sourced middle Cambrian erratics (trilobite shales) support that Cambrian shallow marine sedimentation continued during Wuliuan–?Guzhangian ages in the Shackleton Range sector (Fig. 21). However, the tectonic emplacement of the Mount Wegener nappe and its very low-grade metamorphic overprint is older (around 490 Ma) than the weak tectonic deformation and low-grade metamorphic overprint observed in the trilobite shales (463-455 Ma). This suggests that middle Cambrian shallow marine sedimentation did not develop in the same tectonosedimentary environment (Fig. 21).
10. Palaeoenvironmental reconstruction of the lost Cambrian platform
The analysis of the microfacies of carbonate clasts allows us to reconstruct different sub-environments of the lost carbonate platform from which they were derived. The analysed carbonate clasts belong to different groups of lithofacies (dolomitic sandstones to sandy dolostones, aggregate grain- to ooid-rich dolostones, dolostones, calcimicrobe- and archaeocyath-rich limestones) – an outcome of the carbonate production in shallow waters from platform-interior ?restricted, oolitic shoal complex and open subtidal platform settings (Fig. 22). The high proportion of terrigenous sands in some dolostone lithofacies also suggests a mixed siliciclastic–carbonate platform attached to land.
The pervasive fabric-retentive dolomitisation observed in dolostone clasts (RD1 in Fig. 9a, c, f) could indicate conditions like those observed in present-day dolomites in restricted evaporative shallow-marine to supratidal environments (Tucker & Wright Reference Tucker and Wright1990 and references therein). Recent penecontemporaneous dolomites were found within microbial mats (Vasconcelos et al. Reference Vasconcelos, McKenzie, Bernasconi, Grujic and Tiens1995; Mazzullo Reference Mazzullo2000) and the recognised importance of low-temperature microbially mediated dolomite formation has strengthened in the last decades (Petrash et al. Reference Petrash, Bialik, Bontognali, Vasconcelos, Roberts, McKenzie and Konhauser2017 and references therein). It should be noted that microbially mediated dolomitisation produces small amounts of dolomite in modern shallow-marine sediments compared to other fossil examples (e.g., reflux dolomitisation by mesohaline brines is capable of pervasive dolomitisation of large areas of carbonate platform; see Machel Reference Machel2004). However, DiLoreto et al. (Reference DiLoreto, Bontognali, Al Disi, Al-Kuwari, Williford, Strohmenger, Sadooni, Palermo, Rivers, McKenzie, Tuite and Dittrich2019) have found rhombohedral ordered dolomite in microbial mats in Qatari sabkhas dominated by filamentous anoxygenic photosynthetic bacteria. These authors propose that, in parallel to secular changes in ocean geochemistry, the evolution and structure of microbial mat communities may have favoured the predominant type of carbonate precipitation within the mat. Precambrian dolomites are primarily associated with shallow subtidal to intertidal facies related to microbial deposits, while limestones correspond to deeper waters facies (see discussion in Tucker Reference Tucker1992 and references therein). In Neoproterozoic oceans, the co-occurrence of marine aragonite, high magnesium calcite and dolomite (mimetic dolomitisation and primary cement) suggest extremely high magnesium/calcium ratios and marine anoxia (Hood & Wallace Reference Hood and Wallace2018). Furthermore, microbial sulphate reduction probably triggered precipitation of fibrous dolomites from euxinic porewaters (Hu et al. Reference Hu, Cai, Liu, Pederson, Jiang, Shen and Immenhauser2020). Evaporative conditions in combination with high rates of microbial activity (e.g., anoxygenic photosynthetic bacteria in biofilms) could have promoted the formation of extensive penecontemporaneous dolomites on those ancient microbe-dominated carbonate platforms, as suggested by Daye et al. (Reference Daye, Higgins and Bosak2019). Currently, we do not have any direct evidence of evaporite formation, since trace elements, fluid inclusions, oxygen and carbon isotopic data are not available; therefore, any dolomitisation model (microbial, evaporative, seepage-reflux, meteoric-marine mixing-zone) should be viewed with caution. Thus, the observed mimetic penecontemporaneous dolomitisation (RD1) seems to be a facies-selective process associated exclusively with platform-interior ?restricted and oolitic shoal complex settings, developed in a near-surface and/or shallow burial diagenetic setting.
10.1. Platform-interior ?restricted setting: mixed sandy carbonate peritidal and storm-related deposits
The dolomitic sandstones (Fig. 7a) with beach rock cements are interpreted as mixed sandy carbonate shore/flat deposits. Their very well rounded and sorted fine to medium detrital quartz grains suggest long-term abrasion due to the transport of the wind by saltation and surface creep. Therefore, mixed sandy carbonate shore/flat deposits (7 in Fig. 22) could have been fed by coastal eolian deposits. Silty/sandy dolomicrites with irregular vuggy to channelised cavities filled with clastic fillings (Fig. 7d) could reflect tidal flat sedimentation of mixed sandy carbonates with alternation of low energy (fallout of the suspended load during slack-water periods), exposure and/or burrowing (development of vuggy porosity), and sedimentation of the bed load as clastic fillings (pelletoids, superficial type 1 ooids, sand grains) by the action of current or waves under moderate energy conditions.
Sandy very poorly to moderately sorted dolorudites with a mixture of eroded and redeposited intraclasts (silty/sandy dolomicrites, sandy oolitic-type 2-compound intraclasts, oolitic-, aggregate-grain- and algal peloid-rich dolograinstones), fragments of type 3 oolitic cortices, abraded micritic coated clasts and orange fibrous cement crusts were produced during high-energy events such as strong storms (Fig. 7b, c; 6 in Fig. 22). The mixture of intraclasts can be correlated with alternating processes of sedimentation, erosion, reworking and deposition by waves, tides and/or storms in peritidal areas, where intraformational conglomerates are common (Flügel Reference Flügel2004). Indeed, the silty/sandy dolomicrite intraclasts could be rip-up clasts eroded from muddy and mixed tidal flat areas. Storms could produce the observed mix of ooids (types 1–3) that are derived from different sub-environments. Superficial ooids (type 1), mostly with quartz nuclei, point to sandy pelletal tidal flat environments, while larger micritic concentric ooids (type 2 and type 3) indicate high-energy environments such as oolitic shoals (see below), as suggested by the study of carbonate grain distribution developed by Steinhoff & Strohmenger (Reference Steinhoff and Strohmenger1996) in the Upper Permian Zechstein 2 of Germany. Therefore, the largest ooids were derived from active shoal settings, while the smallest were produced in moderate- to low-energy, platform-interior settings (8 in Fig. 22). In addition, large sandy oolitic (type 2) compound intraclasts (Fig. 7b), derived from oolitic shoals, also involve repeated reworking and sedimentation in storm-influenced platform-interior settings. The scarcity and low diversity of calcimicrobe remains (Proaulopora, Renalcis; Fig. 8d) in the storm-related deposits indicate that they occasionally colonised the platform-interior, while meanwhile a high siliciclastic input prevented the expansion of other calcimicrobes. Proaulopora remains have been described in fenestral, peloidal and microbial grainstones developed in high-energy peritidal settings of the lower Cambrian Láncara Formation, Spain (Álvaro et al. Reference Álvaro, Vennin, Moreno-Eiris, Perejón and Bechstädt2000).
10.2. Oolitic shoal complex
Another important group of carbonate clasts is formed by the aggregate grain- to ooid-rich dolostones. These are dominated by aggregate grains or ooids or a mixture of both, reflecting different but closely related sedimentary sub-environments in a shallow subtidal to intertidal shoal complex (9 in Fig. 22). The low detrital sand content suggests that sedimentation took place far from the influence of coastal siliciclastic input.
Ooid-rich dolostones have noticeable diagenetic and microfacies characteristics linked with their paleoenvironmental settings (e.g., mimetic dolomitisation, concentric micritic laminae, ooid sizes). The type 2–3 ooids of the studied carbonate clasts are laminar concentric dolomicritic ooids (Figs 7f, 9a, c, f). The micritic laminae are considered secondary microfabrics produced by physical-chemical and/or microbial processes. Laboratory experiments suggest that mimetic concentric dolomite ooids may be useful indicators of precursor aragonite ooids and early dolomitisation (Zempolich & Baker Reference Zempolich and Baker1993). The role of agitation and abrasion can be correlated with the size of the ooid, the density of the bands and the cortical fabric (Medwedeff & Wilkinson Reference Medwedeff, Wilkinson and Peryt1983), so equivalent rates of carbonate precipitation and cortex abrasion could produce micritic ooids (Wilkinson et al. Reference Wilkinson, Buczynski and Owen1984). However, micritic laminae have also been explained as micritisation by endolithic cyanobacteria and final filling of microborings with random microcrystalline cement (Margolix & Rex Reference Margolix and Rex1971; Reid & MacIntyre Reference Reid and MacIntyre2000). In addition, research in Bahamian ooids shows that microbes do not play an important role in early ooid genesis, but modify the chemistry and microfabrics of the cortices through extensive microboring activity and biotic aragonite cementation associated with cyanobacteria Solentia sp. and Hyella sp. (Duguid et al. Reference Duguid, Kurtis Kyser, James and Rankey2010). Some Cambrian ooids show different types of microbial activity as well, such as encrusting filamentous cyanobacteria (e.g., Girvanella-cortex ooids; Liu & Zhang Reference Liu and Zhang2012) or the presence of microbial microborings, filaments and extracellular polymeric substances or EPS (Tan et al. Reference Tan, Shi, Tian, Wang and Wang2018).
The selection, composition (type 2–3 ooids) and presence of broken and overgrown ooids indicate that oolitic dolograinstones correspond to highly turbulent environments caused by the action of waves, tides and/or currents that form oolitic shoal deposits. The development of giant ooids (type 3) is favoured by high saturation of seawater carbonate, low supply of nuclei, high accretion rates, high current velocities and ramp-style architecture according to Sumner & Grotzinger (Reference Sumner and Grotzinger1993). Other experiments carried out by Trower et al. (Reference Trower, Lamb and Fischer2017) indicate that both precipitation and abrasion play a significant role in the final size of ooids. They correlated the mode of transport and the size of ooids, so that the transport as suspended load produces larger ooids than those dominated by bed load transport. In the studied carbonate clasts, the different ooids (types 1–3) occur in characteristic microfacies, the small superficial ooids with quartz nuclei (type 1) suggest platform-interior settings with high siliciclastic contribution, while medium and giant ooids correspond to high-energy oolitic shoal settings. The most common examples of giant ooids come from Precambrian, Cambrian, Lower Triassic and Jurassic platforms (Lehrmann et al. Reference Lehrmann, Minzoni, Li, Yu, Payne, Schaal and Enos2012 and references therein). The lower Cambrian Qingxudong Formation, Sichuan Basin, could be a counterexample for giant ooids developed in deepening and moderate-energy subtidal environments affected by episodic hydrodynamic events (Tan et al. Reference Tan, Shi, Tian, Wang and Wang2018).
Those depositional textures with abundant dolomicrite and varying proportions of ooids and/or aggregate grains indicate low- to moderate-energy conditions in the vicinity of oolitic shoal settings. The loosely packed oolitic dolowackestones represent a mixture of reworked ooids (type 3–2) that were redeposited by waves/storms in low-energy, depressed, muddy areas that were adjacent to an oolitic shoal setting, similar to washover deposits (6 in Fig. 22). The aggregate grain dolowackestones and dolopackstones (Fig. 7e) with the finest size sediment represent low-energy, depressed, muddy areas in a protected subtidal backshoal setting. Nowadays, aggregate-grains are characteristic allochems in shallow marine environments on tropical and subtropical carbonate platforms. For instance, the Bahama Banks grapestones are indicative of uneven water turbulence, low sedimentation rates and low-nutrient environments (Illing Reference Illing1954; Purdy Reference Purdy1963a, Reference Purdyb; Winland & Matthews Reference Winland and Matthews1974) developed in the vicinity of the shelf-margin reefs, and grading into oolitic shoals and algal-foraminiferal sands (Flügel Reference Flügel2004 and references therein).
The aggregate grain-oolitic dolograinstone (Fig. 7g) shows a characteristic laminoid fenestral fabric that can result from multiple processes such as degassing of decaying organic matter and desiccation of microbial mats and lime mud, among others, which are commonly associated with modern peritidal environments (Scholle & Ulmer-Scholle Reference Scholle and Ulmer-Scholle2003; Flügel Reference Flügel2004). The lack of quartz sand grains suggests that sedimentation took place far from the coastal siliciclastic inputs. The mixture of components (e.g., aggregate grains, medium and giant ooids, large compound intraclasts) reflects that the fenestral aggregate grain-oolitic dolograinstones developed in transition zones between aggregate-rich areas and active oolitic shoals. Finally, the observed diagenetic vadose fabrics (e.g., intraparticle secondary porosity as oomolds, internal geopetal infills, microcrystalline crust, meniscus cement; Figs 7f, g, 9b) indicate that they developed in intertidal conditions, likely on a partially/episodically subaerially exposed oolitic shoal setting.
10.3. Open subtidal platform setting: calcimicrobe carpets, calcimicrobe–archaeocyath patch reefs and storm-related deposits
The calcimicrobe-rich and/or archaeocyath-rich limestone clasts from the Mount Wegener Formation and Cenozoic erratics evidence different environmental conditions in an open subtidal platform (1–5 in Fig. 22). The co-occurrence of calcimicrobes and archaeocyaths points out photic, normal conditions. As above, the low content of detrital quartz sand grains suggests shallow subtidal conditions far away from the coarse detrital siliciclastic coastal inputs. Calcimicrobe-rich microfacies are derived from calcimicrobial boundstones (calcimicrobe carpets; 1 in Fig. 22) and/or calcimicrobial boundstones with varying proportions of archaeocyaths (calcimicrobe–archaeocyath patch reefs; 2–4 in Fig. 22). The diversity of archaeocyath-rich microfacies shows that the archaeocyaths colonised diverse sub-environments, forming calcimicrobe–archaeocyath patch reefs under varying conditions of water turbulence (calcimicrobe–archaeocyath boundstones and archaeocyath cementstones), and living in open spaces (calcimicrobe-free spaces; 5 in Fig. 22), on muddy substrates that could be disturbed during storms (archaeocyath floatstones; 6 in Fig. 22).
The affinities of calcimicrobes have been a controversial issue; they have been interpreted as cyanobacteria, green and red algae, diagenetic microfossils, fossilised biofilm clusters or fossilised microbial colonies (Pratt Reference Pratt1984; Riding Reference Riding and Riding1991, Reference Riding, Zhuravlev and Riding2001; Stephens & Sumner Reference Stephens and Sumner2002; Woo & Chough Reference Woo and Chough2010 and references therein). However, many of them have been considered mostly fossil calcified cyanobacteria (Riding Reference Riding and Riding1991; Latif et al. Reference Latif, Xiao, Riaz and Hussein2019) and others such as Renalcis and Epiphyton are regarded as algae (Luchinina Reference Luchinina2013 and references therein), as problematic calcified microfossils (Liu et al. Reference Liu, Wu, Jiang, Wu and Jia2017) or as colonies of calcified coccoid cyanobacteria (Zhang et al. Reference Zhang, Dai, Wang and Qi2019). In the Shackleton Range, calcimicrobes clearly linked to filamentous cyanobacteria are Girvanella, Subtifloria (calcified oscillatorialean cyanobacteria) or Proaulopora (calcified nostocalean cyanobacterium) according to Liu et al. (Reference Liu, Liang, Wu, Zhou, Jia and Riding2020).
The occurrence of calcimicrobes in different microfacies of the carbonate clasts allows us to reconstruct their distribution on the platform. Calcimicrobe carpets are dominated by low-diverse calcimicrobial microframeworks. The frequency and prevalence of Epiphyton and/or Angusticellularia suggest that they could easily colonise substrates forming carpets or meadows on the Cambrian seabed, where grazing pressure was low (Debrenne & Zhuravlev Reference Debrenne and Zhuravlev1997). In contrast to Epiphyton and Angusticellularia, Proaulopora and Renalcis appear to have no problem forming carpets/meadows near to siliciclastic inputs. Extensive calcimicrobial colonisation of the substrate may inhibit larval settlement of sessile benthic organisms such as filter-feeding archaeocyaths, where competition for available ‘open space’ is important when grazing pressure is low. The growth rate of archaeocyaths is considered relatively slow so they were easily overgrown by calcimicrobes or buried by mud (Zhuravlev Reference Zhuravlev and Stanley2001 and references therein). In fact, in the Epiphyton-group-dominated bioherms, some archaeocyaths are smaller and have a thick exotheca (Zhuravlev Reference Zhuravlev1996 and references therein).
Calcimicrobe–archaeocyath patch reefs correspond to boundstone microfacies where calcimicrobes were the main framebuilders (except in the archaeocyath cementstone), while sessile heterotrophs such as archaeocyaths and coralomorphs played a minor or passive framebuilder role together with chancelloriids and hyoliths (part of an accessory heterozoan assemblage). The most common framebuilder in Cambrian reefs is Renalcis, encrusting archaeocyath cups, which form Renalcis-archaeocyath boundstones (Debrenne Reference Debrenne2007) or calcimicrobial thrombolytic framestones (type 2 of Gandin & Debrenne Reference Gandin and Debrenne2010). In the Shackleton Range sector, the main calcimicrobes are Epiphyton, Angusticellularia and subordinate Tarthinia, Renalcis and Girvanella, with rare occurrences of Botomaella.
In the Shackleton Range sector, the ajacicyathides proliferated in the open subtidal platform from muddy bottoms to calcimicrobe–archaeocyath patch reefs (see Appendix 1). They played different roles as minor framebuilders in the calcimicrobial boundstones with archaeocyaths or as main framebuilders in the archaeocyath cementstones. In Cambrian ecosystems, the mostly solitary ajacicyathides inhabited soft, muddy substrates with a high sedimentation rate (Debrenne Reference Debrenne2007), as well as forming thickets and skeletal piles for the successive calcimicrobe–archaeocyath colonisation (Zhuravlev Reference Zhuravlev and Stanley2001).
The maximum diversity in the number of archaeocyath families (75 %) and the presence of rare calcimicrobe intergrowths (highest calcimicrobe diversity) occurs in the calcimicrobial boundstone with archaeocyaths (2 in Fig. 22; see Appendix 1). It could represent a transition zone between low-diversity calcimicrobe carpets and other calcimicrobe–archaeocyath patch reefs, where the volume of sessile archaeocyaths increases (from 15 % to 50 %) but the diversity of families decreases (30 % and 35 %, respectively; see 3 and 4 in Fig. 22). Furthermore, the small size of the growth framework cavities in the calcimicrobial boundstone with archaeocyaths indicates a homogeneous and dense colonisation of the substrate by calcimicrobes. In the Shackleton Range, Epiphyton–Tarthinia–Girvanella or Epiphyton–Girvanella intergrowths are not common (Fig. 8f–i). Examples of Cambrian Series 2 intergrowths have been described in the Tarthinia–Epiphyton–Gordonophyton–Renalcis boundstone from Mongolia (Wood et al. Reference Wood, Zhuravlev and Chimed Tseren1993) and in dendritic thrombolites from North China (Lee et al. Reference Lee, Lee, Chen, Woo and Chough2014). However, both examples lack Girvanella intergrowths and the latter lacks co-occurrence with archaeocyaths. Toyonian archaeocyathan-calcimicrobial reefs of South China display a diverse assemblage of calcimicrobes (Adachi et al. Reference Adachi, Nakai, Ezaki and Liu2014), where Girvanella is found intergrowing with Epiphyton bushes and encrusting archaeocyaths. However, Girvanella crusts around archaeocyaths are very rare in the Shackleton Range sector.
The calcimicrobe–archaeocyath patch reefs with a lower diversity of archaeocyathan families (35 %) show larger growth cavities and an extensive development of early marine cement-supported fabrics, indicating that these patch reefs (calcimicrobe–archaeocyath boundstone and archaeocyath cementstone, respectively) developed wave-resistant frameworks under high-energy conditions.
The peloid–intraclastic–bioclastic packstones to grainstones with remains of archaeocyaths could represent storm sheets derived from areas close to the calcimicrobe–archaeocyath patch reefs and surrounding muddy bottoms and deposited above the fair-weather wave base (FWWB), whereas the archaeocyath floatstones were deposited below the FWWB.
The muddy bottoms were colonised by a diverse heterozoan assemblage. Trilobites, brachiopods, hyoliths and echinoderms are common in wackestone to packstone pockets, thus likely living in the vicinity of the calcimicrobe–archaeocyath patch reefs on muddy subtidal substrates. There is a high diversity of archaeocyath families (55 %) in wackestone to floatstone, the presence of Bronchocyathidae, Kymbecyathidae, Dictyocyathidae and Copleicyathidae being exclusive, with Bronchocyathidae being the dominant family together with the conspicuous Ajacicyathidae.
11. Comparison with neighbouring Cambrian inboard successions of the EAC
The Cambrian carbonate platform inboard successions are restricted to the central and southern Transantarctic Mountains and the PM (TAM and PM in Fig. 21) and they record the transition from passive to active margin deposition (Goodge Reference Goodge2020 and references therein).
The best-known Cambrian Series 2 carbonate record corresponds to the lower Atdabanian to Botoman Shackleton Limestone (Byrd Group; Fig. 23 and references therein) in the central TAM (sector c-d in Fig. 21). The total thickness of the unit is unknown due to tectonic complication. The lower mixed siliciclastic–carbonate part is well recognised in the central Holyoake Range on the Errant Glacier side (~133 m thick; Myrow et al. Reference Myrow, Pope, Goodge, Fischer and Palmer2002a, fig. 4). The upper carbonate-dominated succession, around 225–320 m thick, has been analysed in the N and centre of the Holyoake Range (Rees et al. Reference Rees, Pratt and Rowell1989; Claybourn et al. Reference Claybourn, Jacquet, Skovsted, Topper, Holmer, & Brock and A2019, respectively). The lower Shackleton Limestone consists of interbedded quartz sandstone with mud drapes and wave ripples and fine-grained dolomitic grainstone with hummocky cross-stratification (Myrow et al. Reference Myrow, Pope, Goodge, Fischer and Palmer2002a).

Figure 23 Reconstruction of the hidden Cambrian marine record in the Shackleton Range and possible equivalences to nearby Cambrian successions in the Whichaway Nunataks, the Pensacola Mountains (Argentina Range, Neptune Range) and the central Transantarctic Mountains. The most representative ages are given; for the Shackleton Range, see also Fig. 3. Key: 1 = Oldhamia cf. radiata, Oldhamia cf. antiqua, Buggisch et al. (Reference Buggisch, Kleinschmidt, Kreuzer and Krumm1990). 2a = archaeocyath presence, Rb–Sr sedimentation? ages, and 2b = K–Ar metamorphism ages according to Buggisch et al. (Reference Buggisch, Kleinschmidt, Höhndorf and Pohl1994a). 3 = Rb–Sr diagenesis? ages, Pankhurst et al. (Reference Pankhurst, Marsh, Clarkson, Oliver, James and Jago1983). 4 = K–Ar mixtures? of inherited lower Cambrian ages of sedimentation/ diagenesis and Ross metamorphism, Buggisch et al. (Reference Buggisch, Kleinschmidt, Kreuzer and Krumm1994b). 5 = K–Ar detrital muscovites ages with late Pan-African cooling history, Buggisch & Henjes-Kunst (Reference Buggisch and Henjes-Kunst1999). Trilobite-based ages: 6a = Palmer & Gatehouse (Reference Palmer and Gatehouse1972); 6b = Solov'ev & Grikurov (Reference Solov'ev and Grikurov1979); 6c = Cooper & Shergold (Reference Cooper, Shergold and Tingey1991); 6d = Lieberman (Reference Lieberman2004). Brachiopods: 7a = Thomson (Reference Thomson1972); 7b = Notiobulus tenuis, Mayan stage, Popov & Solov'ev (Reference Popov and Solov'ev1981). Archaeocyath presence: 8 = Höfle & Buggisch, (Reference Höfle and Buggisch1995); 9 = Stephenson (Reference Stephenson1966). Archaeocyath-based age: 10a, b, c = Hill (Reference Hill and Adie1964a, Reference Hill1964b, Reference Hill1965); 11 = Konyushkov & Shulyatin (Reference Konyushkov, Shulyatin and Zhuravleva1980); 12 = Debrenne & Kruse (Reference Debrenne and Kruse1989). 13 = Bradoriids, Rode et al. (Reference Rode, Lieberman and Rowell2003). U–Pb detrital zircons ages: 14a = older limit for the depositional age; and 14b = maximum depositional age for the Patuxent Formation (rest.), in a restricted sense, according to Rowell et al. (Reference Rowell, Van Schmus, Storey, Fetter and Evans2001); 15 = youngest zircon grains age and ?early Cambrian maximum depositional age inferred according to Goodge et al. (Reference Goodge, Williams and Myrow2004a). Trilobite-based ages: 16 = Evans et al. (Reference Evans, Rowell and Rees1995); 17 = Evans et al. (Reference Evans, Mckenna, Lieberman, Weichert and Macleod2018). 18 = archaeocyath-based age, Wood et al. (Reference Wood, Evans and Zhuravlev1992). 19 = brachiopods, trilobites, Bassett-Butt (Reference Bassett-Butt2016). 20 = Notiobulus sp., Storey et al. (Reference Storey, Macdonald, Dalziel, Isbell and Millar1996). U–Pb zircon volcanism/magmatism ages: 21 = Millar & Storey, (Reference Millar and Storey1995), Van Schmus et al. (Reference Van Schmus, McKenna, Gonzales, Rowell and Ricci1997); 22 = Goodge et al. (Reference Goodge, Myrow, Williams and Bowring2002). Trilobite-based ages: 23 = Rowell et al. (Reference Rowell, Rees, Cooper and Pratt1988), Palmer & Rowell (Reference Palmer and Rowell1995); 24a = Myrow et al. (Reference Myrow, Pope, Goodge, Fischer and Palmer2002a). 24b = carbon isotope excursion IV (equivalent to CARE), Myrow et al. (Reference Myrow, Pope, Goodge, Fischer and Palmer2002a). 25 = archaeocyath-based ages, Debrenne & Kruse (Reference Debrenne and Kruse1986). 26 = Mollusc-based ages, Claybourn et al. (Reference Claybourn, Jacquet, Skovsted, Topper, Holmer, & Brock and A2019). 27 = brachiopod-based ages, Claybourn et al. (Reference Claybourn, Skovsted, Holmer, Pan, Myrow, Topper and Brock2020). 28a, b, e = detrital youngest zircon grain ages, Goodge et al. (Reference Goodge, Williams and Myrow2004a, Reference Goodge, Myrow, Phillips, Fanning and Williams2004b). 28c, d = U–Pb crystallisation ages, Goodge et al. (Reference Goodge, Myrow, Phillips, Fanning and Williams2004b). 29 = Kruse & Debrenne (Reference Kruse and Debrenne2020). 30 = this study. Abbreviations: MW Fm = Mount Wegener Formation; EAC = East Antarctic craton; WH Fm = Wyeth Heights Formation; SB Fm = Stephenson Bastion Formation; OHT = Otter Highlands Thrust; MWT = Mount Wegener Thrust; GF Mb = Gorecki Felsite Member; PR = Patuxent Range; SSF = small shelly fossils; HF = Holyoake Formation; DC = Douglas Conglomerate; SR = Sedimentary rocks; MR = metamorphic rocks; VR = volcanic rocks; IR = igneous rocks; (*) = Rest of the record not represented. Stages used in each year of publication: Australian stages (Ordian, Templetonian, Floran, Undillan, Boomerangian); Siberian stages (Aldan, Lena, Botoman, Toyonian, Mayan). Precambrian and Cambrian subdivisions used in bold are according to the International Chronostratigraphic Chart (Cohen et al. Reference Cohen, Finney, Gibbard and Fan2013, available online at https://stratigraphy.org/chart).
The upper Shackleton Limestone comprises supratidal (Burgess & Lammerink Reference Burgess and Lammerink1979) to deep subtidal deposits on a carbonate ramp (Myrow et al. Reference Myrow, Pope, Goodge, Fischer and Palmer2002a) where isolated bioherms (<2 m) and biohermal complexes (20–50 m thick) proliferated (Rees et al. Reference Rees, Pratt and Rowell1989). Rees et al. (Reference Rees, Pratt and Rowell1989) recognised four depositional sub-environments whose lithofacies associations share some characteristics with those recognised in the Shackleton Range. The intertidal association corresponds to dolomitic limestones (fenestral cryptomicrobial laminites, fenestral mudstone, parallel- and ripple cross-laminated peloidal grainstone and dolomitic intraclastic flat pebble rudstone), while in the Shackleton Range they correspond to mixed sandy penecontemporaneous dolostones with a diverse allochem association. The carbonate sand shoal association shows varied allochems (ooids, coated grains, oncoids, bioclasts, grapestones) in thick, parallel-, planar cross- and bimodal cross-stratified grainstone sequences, which are interbedded with bioclastic, intraclastic and peloidal grainstones as well as oncolite rudstones. However, oncolite rudstones and bioclastic grainstones are not found in the Shackleton Range sector. In the Shackleton Limestone, the microstructures of ooids are diverse (radial, concentric or composite versus concentric cortices) as well as their nuclei (peloids, trilobites, echinoderms versus quartz grains or broken cortices). However, the presence of giant ooids or sandy oolitic compound intraclasts is not reported in the Shackleton Limestone. And, although early diagenetic processes are recorded (isopachous cement, micritisation, oomoldic and shelter porosity, equant spar), neither mimetic penecontemporaneous dolomitisation nor early silicification processes are described in the Shackleton Limestone. In fact, dolomitisation of the carbonate sand shoal association is described as partial replacement of ooids by large dolomite crystals. The shallow-subtidal shelf association shows some common lithofacies such as peloid, intraclastic, bioclastic packstones to grainstones or oolitic grainstones derived from nearby sand shoals that are interpreted as storm deposits. However, bioturbated bioclastic wackestones and packstones or spongiostromata oncolite packstones are not recorded in the Shackleton Range sector. The archaeocyathan–microbial reef association is interbedded with burrow-mottled and oolitic limestones, and allochems from the carbonate sand shoals are also present in some of the reef complexes. This interdigitisation of facies and components is not observed in boundstone from the Shackleton Range sector. However, the diversity in calcimicrobes is much higher in the Shackleton Range, since only Renalcis, Epiphyton and Girvanella have been reported in the Shackleton Limestone.
Rees et al. (Reference Rees, Pratt and Rowell1989) described three types of reefs, formed by Epiphyton-bearing boundstone, Renalcis boundstone and stromatactis-bearing boundstone. Only the Epiphyton-bearing boundstone, developed in moderately high-energy areas, share some characteristics with the analysed boundstone microfacies. In fact, Epiphyton and Angusticellularia are the main calcimicrobes in calcimicrobe carpets, as well as in calcimicrobe–archaeocyath patch reefs in the Shackleton Range sector. In the Shackleton Limestone, the dominant reef type is Epiphyton-bearing boundstone with subordinate Girvanella and Renalcis. Archaeocyaths comprise one-third of its volume or are absent. This type of reef developed centimetre- to decimetre-scale, open growth-framework cavities colonised by calcimicrobes, lined by marine cement crusts with multi-episodic geopetal infills. However, boundstones of the Shackleton Range sector display millimetre- to centimetre-scale growth-framework cavities and lack multi-episodic geopetal infills (see mesostructure maps in Fig. 22).
In the central Holyoake Range, towards the upper part of the Shackleton Limestone, the biohermal complexes are capped by thick composite bored phosphatic hardgrounds that indicate the terminal drowning of the bioherms due to tectonic subsidence produced by the initiation of orogenesis in this region (Myrow et al. Reference Myrow, Pope, Goodge, Fischer and Palmer2002a). The successive deep-water transgressive Holyoake Formation, with rare trilobites, grades into the shallowing- and coarsening upward Starshot Formation (Fig. 23 and references therein). The Starshot Formation contains late Botoman trilobites at the base (Myrow et al. Reference Myrow, Pope, Goodge, Fischer and Palmer2002a) and clasts from the conglomeratic levels contain archaeocyathan limestone, metamorphic, igneous and volcanic rocks (Laird Reference Laird1963). The overlying Douglas Conglomerate are alluvial fan, marine, braid deltas and associated lacustrine deposits (Rees & Rowell Reference Rees, Rowell, Thomson, Crame and Thomson1991), which contain sandstone and quartzite, eroded folded limestone from the Shackleton Limestone and rare granitoid, rhyolite, basalt, chert and dolomite clasts (Skinner Reference Skinner and Adie1964, Reference Skinner1965; Rees et al. Reference Rees, Girty, Panttja and Braddock1987). Detrital zircons from the upper part of the Douglas Conglomerate indicate a maximum Miaolingian depositional age of around 506 Ma, and the Starshot Formation is cut by Miaolingian–Furongian intrusions with U–Pb crystallisation ages of 504 Ma and 495 Ma (Goodge et al. Reference Goodge, Myrow, Phillips, Fanning and Williams2004b). Therefore, the Byrd Group (Shackleton Limestone, Holyoake and Starshot Formations and Douglas Conglomerate) reflects a shift from the passive carbonate ramp deposition to the onset of supracrustal deformation during the Botoman that produced the drowning of the carbonate ramp, tectonic deformation, uplift and deposition of a widespread clastic wedge (Myrow et al. Reference Myrow, Pope, Goodge, Fischer and Palmer2002a).
In the central Transantarctic Mountains, Goodge (Reference Goodge2020 and references therein) differentiated four main phases in the Cordilleran-type, active continental-margin Ross orogenic belt:
• Early Neoproterozoic passive-margin phase represented by the Beardmore Group;
• Ediacaran–Cambrian Stage 3 (~580–515 Ma) passive to active margin phase: development of early continental-margin volcanism and Cambrian Series 2 carbonate ramp (Shackleton Limestone);
• Cambrian Stage 3–Jiangshanian (~515–490 Ma) synorogenic phase: intra-arc deformation, magmatism, erosion and siliciclastic sedimentation (Holyoake and Starshot Formations) in a forearc setting (c-d in Fig. 21); and
• Jiangshanian–Tremadocian (~490–480 Ma) late orogenic phase: synorogenic succession intruded by post-tectonic magmas; sedimentation of alluvial fan and shallow marine deposits (Douglas Conglomerate and upper Starshot Formation, respectively).
The in situ Cambrian Series 2 carbonate record closest to the Shackleton Range crops out in the Argentina Range, PM (Fig. 23). Rowell et al. (Reference Rowell, Rees and Evans1992a) referred to this 200-m-thick formation informally as ‘Schneider Hills limestone’ and suggested that it may have been part of the same broad carbonate shelf that the Shackleton Limestone deposited on the western passive margin of the E Antarctica basement (Nimrod Group) (c-d in Figs 21, 23). However, infracrustal rocks do not crop at PM and its Cambrian Series 2 deposits are not contiguous with those of the central TAM (Schneider Hills limestone is absent in the closest Neptune Range). In the PM the supracrustal rocks correspond to the Hannah Ridge Formation (Fig. 23). Detrital zircon data from this formation gave an age of latest Neoproterozoic or younger (Rowell et al. Reference Rowell, Van Schmus, Storey, Fetter and Evans2001; Goodge et al. Reference Goodge, Williams and Myrow2004a). This formation underwent two phases of deformation prior to uplift, erosion and deposition of the Miaolingian Nelson Limestone, which contains supracrustal rock clasts with two cleavages in its basal conglomerate (Curtis & Storey Reference Curtis and Storey2003 and references therein). Evans et al. (Reference Evans, Mckenna, Lieberman, Weichert and Macleod2018) suggested that Cambrian Series 2 (and older) limestones may be covered by snow and ice at the Patuxent Range or were even removed by uplift and erosion in the Neptune Range during an early phase of Ross orogeny. Alternatively, the lack of detrital inputs of this age in the succeeding record could also reflect a local palaeotopographic high that avoid the sedimentation during Cambrian Series 2.
Data on the Schneider Hills limestone are scarce and there is no published stratigraphic section. Konyushkov & Shulyatin (Reference Konyushkov, Shulyatin and Zhuravleva1980) described the presence of calcimicrobes (Renalcis, Epiphyton), stromatolites and gave a list of genera and species of archaeocyaths, but they did not figure them. Later, Debrenne & Kruse (Reference Debrenne and Kruse1989) revised the Antarctic Cambrian archaeocyaths and gave the Konyushkov and Shulyatin's assemblage a Botoman age.
Rowell et al. (Reference Rowell, Rees and Evans1992b) described a succession of several hundred meters, with a lower part containing bioclastic (archaeocyath-rich) and oolitic grainstones, bioturbated wackestones and packstones with scattered microbial reefs (0.5–10 m wide, up to 2 m thick). The top of the lower part is rich in camenellan tommotiids (Dailyatia Rowell et al. Reference Rowell, Rees and Evans1992b; Dailyatia icari sp. nov. Claybourn et al. Reference Claybourn, Skovsted, Betts, Holmer, Bassett-Butt and Brock2021), and bradoriide Bicarinella evansi (Rode et al. Reference Rode, Lieberman and Rowell2003) and is overlaid by 200-m-thick Cambrian, massive boundstone with grainstone and burrowed flanking beds. Archaeocyaths are accessory components in the massive boundstone and flanking beds. The succession continues with 150–250-m-thick, thin-bedded bioturbated limestones with scattered small boundstone mounds and a second massive boundstone with small cavities (<1 cm) and flanking beds of alternating grainstone and burrowed packstone. Rowell et al. (Reference Rowell, Rees and Evans1992b) compared the thick Schneider Hills limestone massive boundstones with those of similar size in Virginia, the shelf-margin skeletal algal reefs described by Barnaby & Read (Reference Barnaby and Read1990).
Comparisons between the Shackleton Range and PM Cambrian Series 2 carbonate platforms are limited due to existing data from the PM. The carbonate clast microfacies from the Shackleton Range sector do not exhibit bioclastic (archaeocyath-rich) grainstone, stromatolite or bioturbated wackestones or packstones. Burrowed dolomicrite with sandy infillings occur in the Shackleton Range sector (Fig. 7d) but we have interpreted them as part of the sandy-mixed carbonate tidal flat (7 in Fig. 22). Carbonate microfacies in common between the two regions are boundstone and oolitic grainstone, but they are widespread in the Cambrian Series 2 carbonate platforms. The mesostructure of calcimicrobe–archaeocyath patch reefs from the Shackleton Range sector (Fig. 22) shows cavities larger than those of the Schneider Hills limestone. Therefore, only the comparisons between the archaeocyathan faunas in both sectors (see section 8) make paleobiogeographic sense.
In the PM, the Ross orogeny is characterised by three Miaolingian to ?Ordovician deformation events (Curtis et al. Reference Curtis, Millar, Storey and Fanning2004 and references therein) constrained by radiometric and paleontological data (Fig. 23). The early contractional deformation event is around 505 Ma and produced folding, associated cleavages, emplacement of syntectonic granites (later Sherpan Peak phase) and exhumation of the Hanna Ridge Formation prior to deposition of the Miaolingian Nelson Limestone. It is followed by a middle Ross extensional event characterised by sedimentation of the Nelson Limestone that terminated by bimodal volcanism ca. 500 Ma (Gambacorta Formation). The Nelson Limestone is >400 m thick (Evans et al. Reference Evans, Rowell and Rees1995, Reference Evans, Mckenna, Lieberman, Weichert and Macleod2018) and is Drumian or Drumian to early Guzhangian in age according to paleontological data (Fig. 23 and references therein), so the total thickness was deposited in a 4–5 Myr time interval.
The Weins Formation has an unconstrained post-Gambacorta Formation pre-Neptune Group age. The presence of Notiobolus sp. is recorded in the Weins Formation (Storey et al. Reference Storey, Macdonald, Dalziel, Isbell and Millar1996). This genus is of Wuliuan–Jiangshanian age, which is the combined range of its three extinct species (Popov & Solov'ev Reference Popov and Solov'ev1981; Holmer et al. Reference Holmer, Popov, Koneva and Bassett2001; Popov et al. Reference Popov, Holmer, Hughes, Ghobadi Pour and Myrow2015). Therefore, the Weins Formation has a ?Guzhangian–?Jiangshanian age. Finally, a late Ross contractional event, ?Furongian–?Ordovician (there are no fossils or radiometric dating), produced the moderate deformation and weak cleavage of the previous units and the syntectonic deformation of the basal part of the overlying Neptune Group (Storey et al. Reference Storey, Macdonald, Dalziel, Isbell and Millar1996).
In summary, the spatial and temporal tectonic variability along the palaeo-Pacific margin and the Mozambique seaway margin (sectors c-d and a-b, respectively, in Fig. 21) during the Ross orogeny controlled the development of carbonate platforms. Cambrian Series 2 carbonate platforms, several hundred meters thick with calcimicrobe–archaeocyath bioconstructions, were developed on both margins during tectonic quiescent intervals from Atdabanian to Botoman (TAM) or at least during Botoman (PM and Shackleton Range). In the central TAM, the occurrence of shallow-water mixed siliciclastic–carbonate deposits is reported in the lower Shackleton Limestone, below the positive carbon excursion at the base of the Atdabanian or Stage 3 (Myrow et al. Reference Myrow, Pope, Goodge, Fischer and Palmer2002a); therefore, part of the mixed sedimentation could have started at the end of Terreneuvian in this sector. In the reconstructed lost Cambrian Series 2 mixed carbonate platform of the Shackleton Range sector, the terrigenous influence is interpreted as part of the sandy mixed carbonate tidal flat deposits. Botoman is the age obtained from the assemblage of archaeocyaths in the Shackleton Range (see section 8). However, an older age cannot be ruled out for the development of a mixed siliciclastic–carbonate platform though, due to the existence of Ediacaran–Terreneuvian detrital ages recorded in the slope to basinal deposits of the Mount Wegener Formation (Fig. 24).

Figure 24 Chronostratigraphy of the Ediacaran to Ordovician sedimentary record of the ‘Northern terrane’ from the Shackleton Range sector and the main tectono-metamorphic and magmatic events recorded in the infra- and supracrustal rocks. Abbreviation: Ordv. = Ordovician. Ages are based on the following works: 1 = Zeh et al. (Reference Zeh, Millar, Kroner and Görz1999), Will et al. (Reference Will, Zeh, Gerdes, Frimmel, Millar and Schmädicke2009). 2 = Romer et al. (Reference Romer, Mezger and Schmädicke2009). 3 = Tessensohn et al. (Reference Tessensohn, Kleinschmidt, Talarico, Buggisch, Brommer, Henjes-Kunst, Kroner, Millar and Zeh1999a). 4 = Pankhurst et al. (Reference Pankhurst, Marsh, Clarkson, Oliver, James and Jago1983), Buggisch et al. (Reference Buggisch, Kleinschmidt, Kreuzer and Krumm1994b), Buggisch & Henjes-Kunst (Reference Buggisch and Henjes-Kunst1999). 5 = Solov'ev & Grikurov (Reference Solov'ev and Grikurov1979), Popov & Solov'ev (Reference Popov and Solov'ev1981), Popov et al. (Reference Popov, Holmer, Hughes, Ghobadi Pour and Myrow2015), Cooper & Shergold (Reference Cooper, Shergold and Tingey1991), Lieberman (Reference Lieberman2004). 6 = Buggisch et al. (Reference Buggisch, Kleinschmidt, Kreuzer and Krumm1994b). 7 = Pankhrust et al. (Reference Pankhurst, Marsh, Clarkson, Oliver, James and Jago1983), Buggisch et al. (Reference Buggisch, Bachtadse and Henjes-Kunst1999). 8 = this study.
Some important differences observed in the Shackleton Range sector are the presence of early diagenetic phases such as pervasive mimetic dolomitisation (RD1) and authigenic and diagenetic silicification (Sch, RS1c-m), which are not described in other Cambrian inboard successions. The observed extensive penecontemporaneous dolomitisation can be related with more restrictive conditions in the Antarctic Mozambique seaway margin than in the Antarctic paleo-Pacific margin. The latitudinal differences (see ~520 Ma paleogeographic reconstruction by Merdith et al. Reference Merdith, Collins, Williams, Pisarevsky, Foden, Archibald, Blades, Alessio, Armistead, Plavsa, Clark and Müller2017) and the character of the seaway margin itself could lead to more arid conditions that, along with microbial activity, favoured early dolomitisation. Likewise, in a back-arc setting, the weathering of the volcanic arc and/or its activity could provide large amounts of dissolved silica that would favour near-surface silicification processes in porous microfacies (e.g., diagenetic vadose fabrics of the oolitic shoal complex). In addition to silica-supersaturated pore fluids, near-surface silicification processes require unsaturated carbonate pore fluids and a pH below 9. There are different mechanisms that promote the replacement of carbonates by silica (see Hesse Reference Hesse1989), but it seems that the organic matter oxidation by sulphate-reducing bacteria would be a key reaction for the precipitation of silica in platform sediments (Noble & Van Stempvoort Reference Noble and Van Stempvoort1989).
In the Shackleton Range sector (Figs 21, 24), the synsedimentary brecciation of the mixed siliciclastic–carbonate platform took place during the terminal Stage 3 (~515.5–514.3 Ma by correlation with the Australian data from Betts et al. Reference Betts, Paterson, Jacquet, Andrew, Hall, Jago, Jagodzinski, Preiss, Crowley, Brougham, Mathewson, García-Bellido, Topper, Skovsted and Brock2018) or during the late Atdabanian to lowermost Botoman (~514.45 Ma considering the correlation age suggested by He et al. Reference He, Zhu, Mills, Wyn, Zhuravlev, Tostevin, Pogge von Strandmann, Yang, Poulton and Shields2019) as indicated by the fauna recorded in the platform-derived clasts in the slope and basinal deposits of the Mount Wegener Formation. The first 700 m of thickness of the Mount Wegener Formation were deposited contemporaneously or shortly after platform breakup, as indicated by the successive diagenetic phases observed and their cross-cutting relationships recorded in the carbonate clasts and polymictic conglomerates (see section 6). At the same time, in central TAM, there was the synchronous development of an erosional unconformity, the drowning of the Shackleton carbonate ramp and its burial by siliciclastic sedimentation (Holyoake and lower Starshot Formations), which led to the cessation of carbonate production in this sector (Figs 21, 23).
It is unknown whether the synsedimentary platform brecciation led to the demise of the carbonate production in the Shackleton Range sector. Shallow marine siliciclastic sedimentation (trilobite shales) may have continued during the Wuliuan–?Guzhangian interval in a different tectonosedimentary setting (?African Mozambique seaway margin; a-b in Figs 21, 24). However, Cambrian carbonate production continued in the PM during Miaolingian (Nelson Limestone) and Furongian (subordinate oolitic limestones of the Weins Formation; see Storey et al. Reference Storey, Macdonald, Dalziel, Isbell and Millar1996; Fig. 23).
The lower Starshot Formation (central TAM, Fig. 23) has some similarities with the Mount Wegener Formation, regardless of their differing depositional environments (shoreline to deeper shelf versus slope to basinal deposits) and tectonosedimentary settings (forearc versus back-arc basin). The polymictic conglomerates from both formations reflect erosion of volcanic arcs and carbonate platforms, archaeocyath-bearing clasts included (Myrow et al. Reference Myrow, Pope, Goodge, Fischer and Palmer2002a). The thickness of the Starshot Formation is unknown – up to 3000 m (Laird Reference Laird1963) – and no depositional contacts have been described. It was deposited from shoreline to deep shelf (Laird Reference Laird1963; Laird et al. Reference Laird, Mansergh and Chappell1971) and sandstone tempestite beds were deposited as wave-dominated turbidites (Myrow et al. Reference Myrow, Fischer and Goodge2002b). The basal part of the Starshot Formation is late Botoman in age (Myrow et al. Reference Myrow, Pope, Goodge, Fischer and Palmer2002a). Therefore, the sedimentation of both units could be partially contemporaneous and under synorogenic conditions.
The total thickness of the Mount Wegener Formation is unknown but probably exceeds 1000 m (Buggisch & Henjes-Kunst Reference Buggisch and Henjes-Kunst1999), especially considering its diagenetic evolution. The Mount Wegener Formation underwent various burial diagenetic processes (see section 6) such as dolomitisation (D2–D4) and hydrocarbon migration before undergoing the final tectonic deformation. The latest burial processes recorded correspond to late-fracture-related cementation (LVA and LVB systems), low-grade tectonically-induced plastic and cataclastic fabrics and very low-grade metamorphic overprinting. The late-fracture-related cementation produced the precipitation of very to extremely coarse non-ferroan to slightly ferroan poikilotopic calcite, ferroan saddle dolomite, coarse to very coarse ferroan calcite and varied silica phases such as megaquartz mosaics (Sqtz), quartz overgrowths and chert and microquartz replacements (RS2c-m). The cataclastic fabrics related to the nappe tectonism post-date all the observed cement and replacement phases. The low-grade metamorphic overprint and the southwards transport of the Mount Wegener Nappe occurred around 490 Ma as a result of the Ross (Pan-African) orogeny (Buggisch et al. Reference Buggisch, Kleinschmidt, Kreuzer and Krumm1994b; Fig. 24).
Finally, the Blaiklock Glacier Group (Shackleton Range), the Neptune Group (PM), the Douglas Conglomerate and the upper Starshot Formation (central TAM) represent late to post-orogenic siliciclastic sedimentation during the late Ross orogeny (Figs 23, 24).
12. Summary and conclusions
• In the Shackleton Range, carbonate clasts from the Cenozoic glacial erratics and the Cambrian Mount Wegener Formation exhibit up to 17 types of microfacies (ten dolostone and seven limestone).
• The Cenozoic glacial erratic archaeocyath-bearing clasts come from a local source at the Shackleton Range: the synorogenic upper slope to basinal deposits of the Cambrian Mount Wegener Formation.
• Specifically, the carbonate clasts come from a lost Cambrian Series 2 mixed siliciclastic–carbonate platform that developed in a back-arc basin on the ?Mozambique seaway, related to the final amalgamation between E and W Gondwana during the Ross (Pan-African) orogeny.
• The Shackleton Range archaeocyathan and coralomorph assemblage consist of 34 taxa, it agrees with a terminal Stage 3 for the lost mixed siliciclastic–carbonate platform. An older age cannot be ruled out due to the presence of Ediacaran–Terreneuvian detrital ages in the Mount Wegener Formation.
• Five new genera (Buggischicyathus, Paragnaltacyathus, Shackletoncyathus, Santelmocyathus and Wegenercyathus), six new species (Rotundocyathus glacius, Buggischicyathus microporus, Paragnaltacyathus hoeflei, Shackletoncyathus buggischi, Santelmocyathus santelmoi and Wegenercyathus sexangulae) and a new family Shackletoncyathidae are described.
• The first occurrence of Kaltatocyathus, Rotundocyathus, ?Baikalocyathus, ?Ussuricyathellus, ?Fallocyathus, ?Antoniocoscinus, ?Retecoscinus, Neoloculicyathus, the coralomorph T. kordae and undoubtedly the presence of Cadniacyathus in Antarctica is reported in the Shackleton Range fauna.
• In the reconstructed lost platform, carbonate production was dominated by non-skeletal grains in platform-interior ?restricted and oolitic shoal complex settings, while the open subtidal platform was dominated by diverse calcimicrobes and a secondary to accessory heterozoan assemblage.
• In the platform-interior ?restricted setting, sandy mixed carbonate peritidal deposits were represented by non-skeletal grains such as mud peloids, bahamite peloids, superficial type 1 ooids and very well-rounded and sorted quartz grains. In the oolitic shoal complex, type 2 and type 3 ooids (giant ooids) were produced in high-turbulence conditions, while aggregate grains predominated in low-energy, depressed and protected backshoal settings.
• The main depositional sub-environments in the open subtidal platform were calcimicrobe carpets dominated by Epiphyton and/or Angusticellularia, calcimicrobe–archaeocyath patch reefs and open muddy bottoms. In the calcimicrobe–archaeocyath patch reefs, the archaeocyaths played an accessory (calcimicrobial boundstones with archaeocyaths) to a secondary/primary framebuilder role (calcimicrobe–archaeocyath boundstones and archaeocyath cementstones) and hyoliths and chancelloriids were the common associated fauna. The environs of the patch reefs and open muddy bottoms were colonised by a diverse heterozoan assemblage of solitary archaeocyaths and coralomorphs, echinoderms, brachiopods, trilobites and sponges (as evidenced by their megascleres).
• The diversity in calcimicrobes (Epiphyton, Angusticellularia, Renalcis, Girvanella, Tarthina, Proaulopora, Subtifloria, Botomaella) is the highest reported in the Antarctic Cambrian Series 2 carbonate platforms so far. Renalcis and Proaulopora were able to colonise bottoms influenced by siliciclastic inputs. Only Proaulopora and Subtifloria do not form boundstones and are recorded as allochthonous calcimicrobe remains in grainstones and floatstones.
• The Ajacicyathidae archaeocyaths proliferated in the open subtidal platform from muddy bottoms to calcimicrobe–archaeocyath patch reefs. The greatest richness in archaeocyathan families (75 %) and calcimicrobe genera is recorded in calcimicrobial boundstones with archaeocyaths, where rare calcimicrobial intergrowths of Epiphyton–Tarthinia–Girvanella and/or Epiphyton–Girvanella occur. This study presents the first record of calcimicrobial intergrowths in Antarctic Cambrian Series 2 carbonate platforms. The diversity in archaeocyath families in calcimicrobe–archaeocyath boundstones and archaeocyath cementstones is lower (30 and 35 %, respectively). Densocyathidae, Ajacicyathidae and Kaltatocyathidae (the latter with exclusive presence in calcimicrobe–archaeocyath boundstones) are the dominant families in the calcimicrobe–archaeocyath boundstones, whereas Ajacicyathidae is the only dominant family of the archaeocyath cementstones. The muddy bottoms display the highest richness in skeletal components and the second largest richness in number of archaeocyathan families (55 %). The archaeocyathan families that predominantly colonised muddy bottoms were Bronchocyathidae, Kymbecyathidae, Copleicyathidae and the conspicuous Ajacicyathidae.
• The Shackleton Range fauna shares 21 genera with the Botoman-equivalent Australian assemblages, but only nine species are shared: Tumuliolynthus irregularis, Kaltatocyathus gregarius, Nochoroicyathus hystrix, Nochoroicyathus lawrencei, Thalamocyathus trachealis, Erismacoscinus bilateralis, Coscinoptycta convoluta, Paranacyathus sarmaticus and Archaeopharetra irregularis. Within Antarctica autochthonous assemblages, only two Shackleton Range archaeocyaths are in common with the coeval carbonate platform faunas: Kymbecyathus avius (Shackleton Limestone, TAM) and Thalamocyathus trachealis (Schneider Hills limestone, Argentina Range).
• The affinity between Shackleton Range fauna and assemblages from Permo-Carboniferous tillites is greater. Shackleton Range fauna shares two species and three genera with the EW (Antarctica); two species and one genus with the Falkland Islands (South America); two species and two genera with the Dwyka Group, main Karoo Basin (South Africa); one species and two genera with the Dwyka Group, Aranos Basin (Namibia) and one genus with the Sierras Australes (Argentina).
• The affinity between the Shackleton Range fauna and assemblages from Antarctic Cenozoic deposits is greater, sharing four species and three genera with the King George Island; two species and five genera with the Wichaway Nunataks; and two species and two genera with the Weddel Sea faunas.
• The reconstructed deposits of the hidden/lost Shackleton Range platform are similar to others produced in coeval Cambrian platforms along the paleo-Pacific margin (TAM), but show clear differences (component richness/diversity, diagenetic and tectonically-induced processes) due to its latitudinal situation and tectonosedimentary evolution.
• The lost carbonate platform deposits underwent early marine phreatic and meteoric vadose diagenetic processes before the breakup and synsedimentary brecciation of the platform. Mimetic penecontemporaneous dolomitisation and early authigenic and diagenetic silicification are recorded only in platform-interior ?restricted and oolitic shoal complex deposits. The breakup and downslope transport of the carbonate clast are recorded as breccia fabrics, irregular vugs with crystal silt and angular quartz grain sedimentary infillings, and geopetal infills oriented inconsistently with the growth polarity of calcimicrobe microframeworks.
• At least the first 700 m of synorogenic upper slope to basinal deposits of the Mount Wegener Formation were deposited at the same time or shortly after the platform breakup around ~515.5–514.3 Ma as indicated by the presence of the terminal Stage 3 archaeocyath- and Tabulaconus-bearing platform-derived clasts. The presence of Cambrian Oldhamia ichnospecies could suggest that sedimentation continued up to Wuliuan and even earliest Guzhangian.
• The Mount Wegener Formation underwent various burial processes such as dolomitisation (D2–D4) and hydrocarbon migration prior to late-fracture-related cementation (non-ferroan to slightly ferroan poikilotopic calcite, ferroan saddle dolomite, ferroan calcite, megaquartz mosaics), low-grade tectonically induced plastic fabrics (distorted archaeocyaths, flattened ooids, different types of twin lamellae in late-fracture-related cements) and, finally, cataclastic fabrics that post-dated all observed cementation and replacement phases.
• In Furongian times, very low-grade metamorphic conditions and tectonically induced features developed with the thrusting of the Mount Wegener Nappe over its foreland (EAC) around 490 Ma during the Ross (Pan-African) orogeny (Buggisch et al. Reference Buggisch, Kleinschmidt, Kreuzer and Krumm1994b).
13. Supplementary material
Supplementary material is available online at https://doi.org/10.1017/S1755691022000111.
14. Author contributions
Prof. Dr Werner Buggisch passed away on 6 April 2019 during the preparation of this manuscript. Dr Buggisch participated in four Antarctic expeditions between 1979 and 1995 and made important contributions to the geological knowledge of the Shackleton Range and the Ellsworth Mountains. We asked Dr Buggisch for the possibility of studying the carbonate and calcimicrobe–archaeocyath-bearing clasts from the Shackleton Range. Dr Buggisch responded enthusiastically and sent us all thin-sections and some samples. Unfortunately, although he was aware of our progress, he died before the manuscript was published. This work could not have been done without his help. Dr Buggisch reviewed and provided critical comments on sections 1, 2 and 3. M. R.-M. drafted sections 1–3, 5–6, 9–11 and designed the corresponding figures. A. P., S. M. and E. M.-E. drafted sections 7 and 8 and designed the corresponding figures and supplementary material. All authors discussed the results, wrote sections 4 and 12 and reviewed the final manuscript.
15. Acknowledgements
We want to thank the work done by Carlos Alonso Recio with the photography of archaeocyaths and montage of the plates (Photography Lab, Paleontology Area, Universidad Complutense de Madrid, UCM). We thank Fernando Cebrián for the graphic design of Shackletoncyathus buggischi. Assistance with the references provided by Pedro Martín Duque was greatly appreciated (Library, Faculty of Geology, UCM). We would like to extend our thanks to Aitor Antón, Juan Carlos Salamanca and Beatriz Moral for their work with the samples (Thin Section Lab, Stratigraphy Area, UCM). This paper is a contribution to the Spanish project CGL2017-87631-P. We especially want to thank all the reviewers for their thorough and accurate comments and recommendations. We would also like to acknowledge the corrections and comments made by Alistair John MacGowan and Iván Moreno González. Thanks to your help this work has improved substantially.