Carnitine (l-3-hydroxy-4-N-N-N-trimethylaminobutyrate) is an essential metabolite that has a number of indispensable functions in intermediary metabolism. The most prominent function lies in its role in the transport of activated long-chain fatty acids from the cytosol to the mitochondrial matrix where β-oxidation takes placeReference McGarry and Brown1–Reference Steiber, Kerner and Hoppel3. All tissues that use fatty acids as a fuel source require carnitine for normal function. Carnitine is derived from dietary sources and endogenous biosynthesisReference Rebouche and Seim4. Carnitine biosynthesis involves a complex series of reactions involving several tissuesReference Hoppel and Davis5. Lysine provides the carbon backbone of carnitine. Lysine in protein peptide linkages undergoes methylation of the ε-amino group to yield trimethyllysine, which is released upon protein degradation. Muscle is the major source of trimethyllysine. The released trimethyllysine is further oxidised to butyrobetaine by the action of trimethyllysine dioxygenase, 3-hydroxy-N-trimethyllysine aldolase and 4-N-trimethylaminobutyraldehyde dehydrogenase. Butyrobetaine is hydroxylated by γ-butyrobetaine dioxygenase to form carnitine. The last reaction which is rate-limiting for carnitine synthesis occurs primarily in the liver and kidneysReference Vaz and Wanders6 (see Fig. 1).
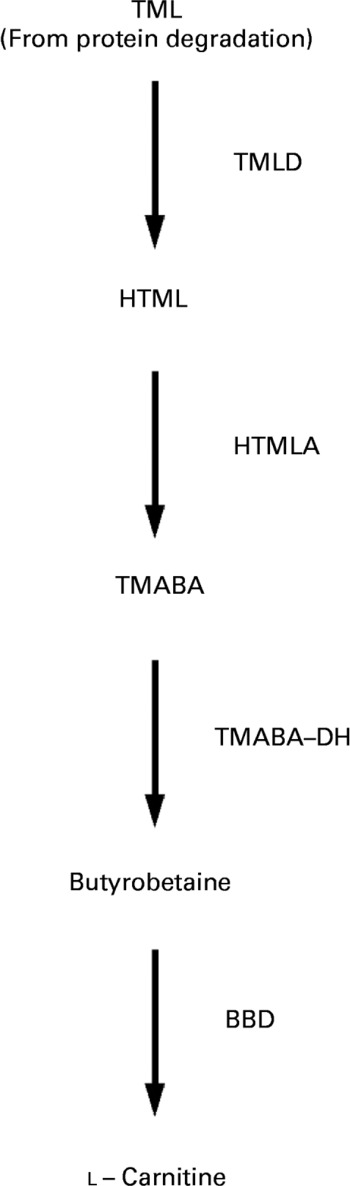
Fig. 1 Schematic diagram of carnitine biosynthesis from trimethyllysine (TML) (according to Vaz & WandersReference Vaz and Wanders6). TML is oxidised to butyrobetaine by trimethyllysine dioxygenase (TMLD), 3-hydroxy-N-trimethyllysine aldolase (HTMLA) and 4-N-trimethylaminobutyraldehyde dehydrogenase (TMABA-DH). In the last rate-limiting step, butyrobetaine is hydroxylated to l-carnitine by γ-butyrobetaine dioxygenase (BBD). HTML, 3-hydroxy-N-trimethyllysine; TMABA, 4-N-trimethylaminobutyraldehyde.
Distribution of carnitine within the body and intracellular homeostasis of carnitine are controlled by organic cation transporters (OCTN) which belong to the solute carrier (SLC) 22A family, localised to the apical membrane of cellsReference Lahjouji, Mitchell and Qureshi7, Reference Tein8. Three OCTN have been identified so far: OCTN1, OCTN2 and OCTN3Reference Tamai, Yabuuchi, Nezu, Sai, Oku, Shimane and Tsuji9–Reference Tamai, Ohashi, Nezu, Sai, Kobayashi, Oku, Shimane and Tsuji11. OCTN are polyspecific; they transport several cations and l-carnitineReference Ohashi, Tamai, Yabuuchi, Nezu, Oku, Sai, Shimane and Tsuji12, Reference Ohashi, Tamai, Nezu Ji, Nikaido, Hashimoto, Oku, Sai, Shimane and Tsuji13. Carnitine transport by OCTN1 and OCTN2 is Na dependent whereas that by OCTN3 is Na independentReference Tamai, Ohashi, Nezu, Sai, Kobayashi, Oku, Shimane and Tsuji11. OCTN1 and OCTN2 are expressed in several tissues such as kidney, intestine, skeletal muscle, heart, liver and brainReference Tamai, Ohashi, Nezu, Sai, Kobayashi, Oku, Shimane and Tsuji11, Reference Wu, Huang, Prasad, Seth, Rajan, Leibach, Chen, Conway and Ganapathy14, Reference Slitt, Cherrington, Hartley, Leazer and Klaassen15. In contrast, OCTN3 is expressed exclusively in the testes and kidneysReference Tamai, Ohashi, Nezu, Sai, Kobayashi, Oku, Shimane and Tsuji11. Among the three OCTN, OCTN3 has the highest specificity for carnitine; OCTN1 has the lowest oneReference Tamai, Ohashi, Nezu, Sai, Kobayashi, Oku, Shimane and Tsuji11. OCTN operate on the intestinal absorption and renal reabsorption of carnitine and play a major role in tissue distribution by catalysing the uptake of carnitine into body cells. Due to its high binding affinity for carnitine and its wide expression, OCTN2 seems to be the most physiologically important carnitine transporter. OCTN1 contributes less to carnitine transport than OCTN2 due to its low carnitine transport activity. OCTN3 may be important for carnitine uptake into the testes, and may contribute to the reabsorption of carnitine in the kidneysReference Tamai, Ohashi, Nezu, Sai, Kobayashi, Oku, Shimane and Tsuji11. The fact that inborn or acquired defects of OCTN lead to primary or secondary systemic carnitine deficiency demonstrates their essential role in carnitine homeostasisReference Tein8.
It has been shown previously that starvation or treatment of rats with clofibrate increases the concentration of carnitine in the liverReference McGarry, Robles-Valdes and Foster16–Reference Paul and Adibi18. Both starvation and clofibrate treatment lead to an activation of PPARα, a transcription factor belonging to the nuclear hormone receptor superfamilyReference Schoonjans, Peinado-Onsurbe, Lefebvre, Heyman, Briggs, Deeb, Staels and Auwerx19. We have recently shown that activation of PPARα by clofibrate treatment causes an up regulation of OCTN1 and OCTN2 in rat liverReference Luci, Geissler, König, Koch, Stangl, Hirche and Eder20. These results strongly indicated that increased carnitine concentrations in livers of rats starved or treated with clofibrate were due to increased uptake of carnitine from blood into the liver. Indeed, plasma carnitine concentrations were reduced in rats treated with clofibrate which may be caused by an increased uptake into the liverReference Luci, Geissler, König, Koch, Stangl, Hirche and Eder20.
In addition to synthetic agonists, several naturally occurring compounds are able to activate PPARα in vivo. Recently, we and others have shown that dietary oxidised fats prepared by the heating of vegetable oils activate hepatic PPARα in rats and pigsReference Chao, Chao, Lin and Huang21–Reference Luci, König, Giemsa, Huber, Hause, Kluge, Stangl and Eder25. In the present study, we tested the hypothesis that oxidised fats are also able to up regulate the expression of OCTN (OCTN1, OCTN2) in the liver due to their ability to activate PPARα and thereby increase hepatic carnitine concentration. For this end, we performed an experiment with growing rats as an animal model, according to a previous study dealing with the effects of an oxidised oil on PPARα activationReference Sülzle, Hirche and Eder24.
More than 95 % of the total carnitine in the body is localised in the muscle which serves as a carnitine storageReference Brass2. When plasma carnitine concentrations are lowered, such as by treatment with pivalate, carnitine is mobilised from the muscle in order to normalise plasma carnitine concentrationsReference Nakajima, Kodo, Inoue, Kizaki, Nukina, Kinugasa and Sawada26. Therefore, an increased uptake of carnitine from the blood into the liver by up regulation of hepatic OCTN should lead to a mobilisation of carnitine storage in the muscle. To investigate this, we also determined carnitine concentrations in skeletal muscle and heart of the rats.
OCTN1 and OCTN2 are also highly expressed in the intestine and particularly OCTN2 plays an important role in the absorption of l-carnitine from the dietReference Slitt, Cherrington, Hartley, Leazer and Klaassen15, Reference Taylor27, Reference Kato, Sugiura, Sugiura, Wakayama, Kubo, Kobayashi, Sai, Tamai, Iseki and Tsuji28. As the small intestine also has a high expression of PPARα, it seems possible that an oxidised fat could increase the gene expression of OCTN also in the small intestine via an activation of PPARα. Besides OCTN, the amino acid transporter ATB0+ is involved in the intestinal absorption of carnitine from the dietReference Taylor27, Reference Nakanishi, Hatanaka, Huang, Prasad, Leibach, Ganapathy and Ganapathy29. In order to obtain information whether PPARα activation by synthetic or native agonists could influence intestinal carnitine absorption, we also determined mRNA concentration of ATB0+ in small intestine.
Materials and methods
Animal experiment
Male Sprague–Dawley rats, aged 5 weeks old, supplied by Charles River (Sulzfeld, Germany) with an average initial body weight of 115 (sd 14) g were randomly assigned to two groups of nine rats each. They were kept individually in Macrolon cages in a room controlled for temperature (22 ± 2°C), relative humidity (50–60 %) and light (12 h light–dark cycle). All experimental procedures described followed established guidelines for the care and handling of laboratory animals and were approved by the council of Saxony-Anhalt. The animals received either 2 ml fresh sunflower-seed oil (control group) or oxidised sunflower-seed oil (see Preparation of the oxidised fat) by oral administration once per d 2 h after the beginning of the light cycle. Afterwards, they obtained their daily food ration. All rats were fed a commercial standard basal diet (Altromin 1324; Altromin GmbH, Lage, Germany). Concentration of total carnitine in the basal diet was 22 μmol/kg. To standardise food intake, diet intake was controlled. Each rat in the experiment received 12 g diet/d. This amount of diet which is approximately 20 % below the amount of diet rats would consume ad libitum was completely ingested by all rats. Thus, the diet intake was identical in all the rats within this experiment. Water was available ad libitum from nipple drinkers during the whole experiment. At day 6 of treatment, rats received the last dose of fresh or oxidised fat and 9 g diet and were killed 4 h later by decapitation under light anaesthesia with diethyl ether. Blood was collected into heparinised polyethylene tubes. Liver, heart and gastrocnemius muscles were quickly removed, frozen with liquid N2 and stored at − 80°C pending further analysis. Plasma was obtained by centrifugation of the blood (1100 g; 10 min; 4°C) and stored at − 20°C. Liver samples for RNA isolation and lipid extraction were snap-frozen in liquid N2 and stored at − 80°C. The small intestine was rapidly excised, washed with cold 0·9 % NaCl (w/v) and mucosal scrapings were obtained from the jejunum (defined by length), snap-frozen and stored at − 80°C for RNA extraction.
Preparation of the oxidised fat
The thermoxidised oil was prepared by heating sunflower-seed oil obtained from a local supermarket in an electric fryer (Saro Gastro-Products GmbH, Emmerich, Germany) for 25 d at 60°C. Throughout the heating process, air was continuously bubbled into the fat. The extent of lipid peroxidation was determined by assaying the peroxide value30, concentration of thiobarbituric acid-reactive substancesReference Sidwell, Salwin, Benca and Mitchell31 and conjugated dienesReference Recknagel and Glende32, acid values30, the percentage of total polar compounds33 and the concentration of total carbonylsReference Endo, Li, Tagiri-Endo and Fujimoto34. The oxidised fat had much higher concentrations of peroxides (126-fold), conjugated dienes (>2740-fold), thiobarbituric acid-reactive substances (12-fold), total carbonyls (33-fold), polar compounds (5-fold) and a higher acid value (15-fold) than the fresh fat (Table 1).
Table 1 Concentrations of various lipid oxidation products in the fats*
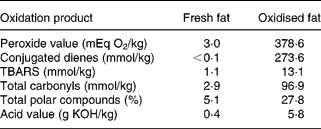
TBARS, thiobarbituric acid-reactive substances.
* Data are the results of single measurements.
Carnitine analysis
Carnitine was determined as [3H]acetyl-carnitine after the esterification of non-esterified carnitine by carnitine acyltransferase according to McGarry & FosterReference McGarry and Foster35 with modifications proposed by Parvin & PandeReference Parvin and Pande36 and Christiansen & BremerReference Christiansen and Bremer37. Plasma samples were used directly for the determination of the total carnitine after alkaline hydrolysation as described for the tissue samples below. Tissue samples were freeze dried and milled. Then 100 mg liver or 50 mg muscle powder were sonificated in 5 ml water for 15 min. Samples were centrifuged (12 000 g; 5 min) and non-esterified carnitine in the supernatant fraction was measured. For the determination of the total carnitine the samples were hydrolysed before the centrifugation. For this, 10 ml 0·2 m-potassium hydroxide were added, the samples were incubated at 30°C for 1 h and then neutralised by the addition of 0·2 m-HCl. Carnitine esterification was done in a final volume of 1 ml containing 0·1 m-HEPES (pH 7·4), 2 mm-N-ethylmaleimide, 1·25 mm-EDTA, 25 μm-[3H]acetyl-CoA (29·4 MBq/mmol; GE Healthcare, Buckinghamshire, UK) and 1 U carnitine acyltransferase (Roche Diagnostic, Mannheim, Germany) for 30 min at room temperature. [3H]acetyl-CoA not consumed by the reaction was bound to Dowex 1-X 8 and separated by centrifugation. Carnitine concentration was calculated using the radioactivity of the supernatant fraction measured in a liquid scintillation counter and corrected for non-specific radioactivity.
Reverse transcriptase polymerase chain reaction analysis
Total RNA was isolated from rat livers and mucosa scrapings, respectively, by TRIZOL™ reagent (Sigma-Aldrich, Steinheim, Germany) according to the manufacturer's protocol. cDNA synthesis was carried out as describedReference König and Eder38. The mRNA concentration of genes was measured by real-time detection PCR using SYBR® Green I and the Rotor Gene 2000 system (Corbett Research, Mortlake, Australia). Real-time detection PCR was performed with 1·25 U Taq DNA polymerase (Promega, Mannheim, Germany), 500 μm-dNTP and 26·7 pmol of the specific primers (Operon Biotechnologies, Cologne, Germany; Table 2). Annealing temperature for all primers was 60°C. For determination of mRNA concentration a threshold cycle (Ct) and amplification efficiency was obtained from each amplification curve using the software RotorGene 4·6 (Corbett Research). Calculation of the relative mRNA concentration was made using the ΔΔCt method as previously describedReference Pfaffl39. The housekeeping gene glyceraldehyde-3-phosphate dehydrogenase was used for normalisation. mRNA concentration of glyceraldehyde-3-phosphate dehydrogenase was not influenced by the treatment of rats with oxidised fat.
Table 2 Characteristics of the primers used in reverse transcriptase polymerase chain reaction analysis
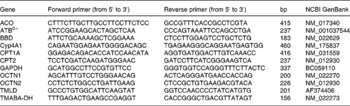
ACO, acyl-CoA oxidase; BBD, γ-butyrobetaine dioxygenase; Cyp, cytochrome P450; CPT, carnitine palmitoyltransferase; GAPDH, glyceraldehyde-3-phosphate dehydrogenase; OCTN, organic cation transporter; TMLD, trimethyllysine dioxygenase; TMABA-DH, 4-N-trimethylaminobutyraldehyde dehydrogenase.
Statistical analysis
Means of the treatment and control groups were compared by an unpaired t test using the Minitab Statistical Software (Minitab, State College, PA, USA). Differences with P < 0·05 were considered to be significant.
Results
Final weights and body-weight gains of the rats
Final body weights of rats treated with the oxidised fat (133 (sd 14) g) were not significantly different from the control rats (144 (sd 14) g) (nine rats for each group). However, rats treated with the oxidised fat had a lower body-weight gain (17·5 (sd 6·4) g) over the feeding period than the control rats (29·7 (sd 4·5) g) (nine rats for each group; P < 0·05).
mRNA concentrations of acyl-CoA oxidase, cytochrome P450-4A1, carnitine palmitoyltransferases-1A and -2, organic cation transporters-1 and -2 and enzymes involved in hepatic carnitine synthesis (trimethyllysine dioxygenase, 4-N-trimethylaminobutyraldehyde dehydrogenase and γ-butyrobetaine dioxygenase) in the liver
Rats treated with the oxidised fat had higher mRNA concentrations of acyl-CoA oxidase, cytochrome P450 (Cyp)-4A1, carnitine palmitoyltransferase (CPT)-2, OCTN1 and OCTN2 in the liver than control rats (P < 0·05); mRNA concentration of CPT1A, however, was not different in the rats treated with oxidised fat from the control rats (Fig. 2). Rats treated with the oxidised fat had a higher mRNA concentration of trimethyllysine dioxygenase in the liver than control rats (P < 0·05; Fig. 2). mRNA concentrations of 4-N-trimethylaminobutyraldehyde dehydrogenase and γ-butyrobetaine dioxygenase in the liver, however, did not differ between the two groups of rats (Fig. 2).
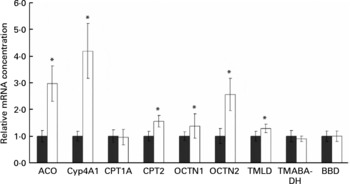
Fig. 2 Effect of an oxidised fat on the relative mRNA concentrations of acyl-CoA oxidase (ACO), cytochrome P450 (Cyp)-4A1, carnitine palmitoyltransferases (CPT)-1A and -2, organic cation transporters (OCTN)-1 and -2, trimethyllysine dioxygenase (TMLD), 4-N-trimethylaminobutyraldehyde dehydrogenase (TMABA-DH) and γ-butyrobetaine dioxygenase (BBD) in the liver of rats. Rats were treated orally with 2 ml oxidised fat (□) or fresh fat (■; control = 1·00) for 6 d. Total RNA was extracted from rat livers and mRNA concentrations were determined by real-time detection RT-PCR analysis using glyceraldehyde-3-phosphate dehydrogenase mRNA concentration for normalisation. Values are means, with standard deviations represented by vertical bars (n 9). *Mean value was significantly different from that of the control rats (P < 0·05).
mRNA concentrations of acyl-CoA oxidase, cytochrome P450-4A1, carnitine palmitoyltransferases-1A and -2, organic cation transporters-1 and -2 and ATB0+ in the small intestine
Rats treated with oxidised fat had higher mRNA concentrations of acyl-CoA oxidase, Cyp4A1, CPT1A, CPT2 and OCTN2 (P < 0·05), and they tended to have a higher mRNA concentration of OCTN1 (P = 0·066) in the small intestine compared with control rats (Fig. 3). mRNA concentration of ATB0+ in the small intestine was reduced in the rats fed the oxidised fat compared with those fed the fresh fat (P < 0·05; Fig. 3).
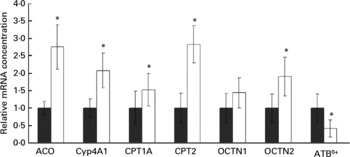
Fig. 3 Effect of an oxidised fat on the relative mRNA concentrations of acyl-CoA oxidase (ACO), cytochrome P450 (Cyp)-4A1, carnitine palmitoyltransferases (CPT)-1A and -2, organic cation transporters (OCTN)-1 and -2 and amino acid transporter ATB0+ in the small intestine of rats. Rats were treated orally with 2 ml oxidised fat (□) or fresh fat (■; control = 1·00) for 6 d. Total RNA was extracted from mucosal scrapings and mRNA concentrations were determined by real-time detection RT-PCR analysis using glyceraldehyde-3-phosphate dehydrogenase mRNA concentration for normalisation. Values are means, with standard deviations represented by vertical bars (n 9). *Mean value was significantly different from that of the control rats (P < 0·05).
Carnitine concentrations in liver, plasma and muscle
Rats treated with the oxidised fat had a higher carnitine concentration in the liver than control rats (P < 0·05, Fig. 4). Plasma carnitine concentration was lower in the rats treated with oxidised fat (18·8 (sd 3·1) μmol/l) than in the control group (28·4 (sd 4·3) μmol/l) (nine rats for each group; P < 0·05). Rats treated with oxidised fat also had lower carnitine concentrations in gastrocnemius and heart muscle than control rats (P < 0·05; Fig. 4).
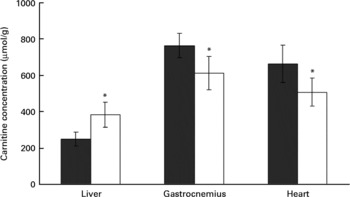
Fig. 4 Effect of an oxidised fat on the concentrations of total carnitine in liver, gastrocnemius and heart. Rats were treated orally with 2 ml oxidised fat (□) or fresh fat (■; control) for 6 d. Values are means, with standard deviations represented by vertical bars (n 9). *Mean value was significantly different from that of the control rats (P < 0·05).
Discussion
We have recently found that treatment with clofibrate causes a strong up regulation of OCTN2, and a less strong up regulation of OCTN1, in the liver of rats which was accompanied by an increased hepatic carnitine concentrationReference Luci, Geissler, König, Koch, Stangl, Hirche and Eder20. This effect was probably caused by PPARα activation. In the present study, we investigated the hypothesis that oxidised fats are able to exert similar effects due to their ability to activate PPARα. Hydroxy- and hydroperoxy fatty acids such as hydroxyoctadecadienoic and hydroperoxyoctadecadienoic acid occurring in oxidised fats are very potent PPARα agonistsReference König and Eder38, Reference Delerive, Furman, Teissier, Fruchart, Duriez and Staels40, Reference Mishra, Chaudhary and Sethi41. These fatty acids are produced during the early stage of lipid peroxidation. Since they are unstable and decompose at high temperatures, fats treated at low temperature have much higher concentrations of these primary lipid peroxidation products than fats treated at high temperatureReference Sülzle, Hirche and Eder24. This is the reason why we used a fat treated at a relatively low temperature for a long period. The high peroxide value and the high concentration of conjugated dienes indicate that this fat indeed had high concentrations of hydroxy- and hydroperoxy fatty acids which may be particularly responsible for the PPARα-activating effects of oxidised fats. To ensure that all rats obtained the same dose of oxidised fat, it was administered orally. The oxidised and fresh fat, respectively, accounted for about 25 % of total energy of the total daily feed. Since it was observed in a previous rat study that even short-term application of a PPARα agonist led to the typical changes known for PPARα activation such as up regulation of classical target genes involved in β-oxidation and reduction of TAG concentrationReference König and Eder38, we decided to give the oxidised fat over a relatively short time of 6 d. It has been shown that the intake of oxidised fats could cause a reduction of the food intake in rats which could cause secondary effects which interact with the effect of treatmentReference Yoshida and Kajimoto42, Reference Liu and Huang43. To ensure an identical food intake in both groups of rats, we used a controlled feeding system in which each rat consumed 12 g diet /d. This amount of diet is slightly below that that rats would consume ad libitum but in clear excess of that necessary to meet the maintenance energy requirement (which is approximately 6 g/d44) and ensures an adequate growth of the rats. It is known that fasting causes an activation of PPARα due to the release of NEFA from the adipose tissueReference Mandard, Müller and Kersten45. To avoid PPARα activation due to an insufficient supply of energy, all the rats received their last portion of diet 4 h before decapitation. Therefore, we can exclude the possibility that PPARα was also activated in the control group fed the fresh fat. The finding that rats fed the oxidised fat gained less weight during the experimental period than those fed the fresh fat although both groups received an identical amount of diet indicates that the oxidised fat impaired the feed conversion ratio. This finding agrees with other reports which also showed that feeding of oxidised fats impairs the growth of ratsReference Corcos Benedetti, D'Aquino, Di Felice, Gentili, Tahliamonte and Tomassi46–Reference Hochgraf, Mokady and Cogan49. We did not investigate the reason for this. Previous studies, however, have shown that oxidised fats lower the digestibility of nutrientsReference Corcos Benedetti, D'Aquino, Di Felice, Gentili, Tahliamonte and Tomassi46, Reference Borsting, Engberg, Jakobsen, Jensen and Anderson50 and this may be the reason for the reduced body-weight gains of the rats fed the oxidised fat observed in the present study. However, as rats fed the oxidised fat appeared quite normal, we assume that the oxidised fat did not cause general toxicity.
The finding of increased mRNA concentrations of the typical PPARα downstream genes acyl-CoA oxidase, Cyp4A1, CPT1A and CPT2 (for a review, see Mandard et al. Reference Mandard, Müller and Kersten45) in liver and intestine indeed indicates that the oxidised fat caused an activation of PPARα in both liver and intestine of the rats. This indication agrees with recent studies in rats and pigs which also showed that intake of oxidised fats leads to an activation of PPARα in the liverReference Chao, Chao, Lin and Huang21–Reference Luci, König, Giemsa, Huber, Hause, Kluge, Stangl and Eder25.
The present study shows further that treatment of rats with an oxidised fat caused the same alterations as observed for clofibrateReference Luci, Geissler, König, Koch, Stangl, Hirche and Eder20, namely increased hepatic mRNA concentrations of OCTN1 and OCTN2 and an increased hepatic carnitine concentration. Considering that a similar up regulation of OCTN1 and OCTN2 was observed in the liver of rats treated with the synthetic PPARα agonist clofibrate and in rat hepatoma cells treated with the more potent and selective PPARα agonist WY 14,643Reference Luci, Geissler, König, Koch, Stangl, Hirche and Eder20, we propose that the oxidised fat up regulated OCTN in the liver also by PPARα activation.
In rat liver, OCTN1 and OCTN2 are highly expressedReference Slitt, Cherrington, Hartley, Leazer and Klaassen15. Both of them are able to transport carnitine into the liver cellReference Yokogawa, Miya, Tamai, Higashi, Nomura, Miyamoto and Tsuji51, Reference Yabuuchi, Tamai, Nezu, Sakamoto, Oku, Shimane, Sai and Tsuji52. However, it has been shown that OCTN2 has a higher carnitine transport activity than OCTN1Reference Tamai, Ohashi, Nezu, Sai, Kobayashi, Oku, Shimane and Tsuji11. For that reason and as mRNA concentration of OCTN2 was more strongly increased by the oxidised fat than that of OCTN1, we assume that increased hepatic carnitine concentrations in rats treated with oxidised fat were caused mainly by an increased uptake of carnitine via OCTN2. Plasma carnitine concentrations are regulated by several events, namely intestinal absorption from the diet, renal excretion, endogenous synthesis in the liver and kidneys and movement of carnitine between plasma and tissuesReference Evans and Fornasini53. We have not studied the pharmacokinetics of carnitine but it seems plausible that reduced plasma concentrations of carnitine in rats fed the oxidised fat may at least in part be due to an enhanced uptake into the liver. We measured mRNA concentrations of OCTN only in liver and small intestine; however, it is possible that they were increased also in other tissues in rats fed the oxidised fat. Therefore, an increased uptake of carnitine into other tissues besides liver could also contribute to the reduced plasma carnitine concentrations. In the kidney, OCTN2 functions to reabsorb carnitine from the urineReference Ohashi, Tamai, Nezu Ji, Nikaido, Hashimoto, Oku, Sai, Shimane and Tsuji13, Reference Tamai, China, Sai, Kobayashi, Nezu, Kawahara and Tsuji54. An up regulation of OCTN2 in kidney would be expected to reduce urinary excretion of carnitine which in turn results in an increased plasma carnitine concentration. However, the effect of oxidised fats on the gene expression of OCTN in those tissues and their consequences on whole-body carnitine homeostasis should be determined in future studies.
In the present study we also determined mRNA concentrations of various enzymes involved in hepatic carnitine biosynthesis in the liver which belongs like the kidney to the tissues being able to synthesise carnitineReference Vaz and Wanders6. It was found that oxidised fat treatment led to a moderate up regulation of trimethyllysine dioxygenase while mRNA concentrations of 4-N-trimethylaminobutyraldehyde dehydrogenase and γ-butyrobetaine dioxygenase, the rate-limiting enzyme of carnitine biosynthesisReference Vaz and Wanders6, remained unchanged by the treatment. This finding shows that PPARα activation by the oxidised oil does not up regulate the gene expression of enzymes involved in hepatic carnitine synthesis. Nevertheless, it is possible that carnitine hepatic biosynthesis was increased in rats treated with oxidised fat. The liver has a high capacity to convert γ-butyrobetaine into carnitineReference Vaz and Wanders6. As OCTN2 has a high affinity for γ-butyrobetaineReference Tamai, Ohashi, Nezu, Yabuuchi, Oku, Shimane, Sai and Tsuji10, Reference Tamai, Ohashi, Nezu, Sai, Kobayashi, Oku, Shimane and Tsuji11 it is likely that an increased expression of OCTN2 may have led to an increased uptake of γ-butyrobetaine from plasma into the liver which in term may have stimulated synthesis of carnitine in the liver. This assumption, however, has to be proven in further studies.
Muscle contains more than 95 % of whole-body carnitine and serves as a carnitine storageReference Brass2. When plasma carnitine concentrations are lowered, such as by treatment with pivalate, carnitine can be mobilised from the muscle in order to normalise plasma carnitine concentrationsReference Nakajima, Kodo, Inoue, Kizaki, Nukina, Kinugasa and Sawada26. Therefore, we expected that a reduced plasma carnitine concentration may lead to a reduction of the carnitine concentration in muscle. The finding that the concentration of carnitine was reduced in gastrocnemius and heart muscle of rats treated with oxidised fat indeed suggests that carnitine might have been mobilised from muscle. In rats treated with clofibrate, a reduction of muscle carnitine concentration has also been foundReference Luci, Geissler, König, Koch, Stangl, Hirche and Eder20. A reduced carnitine concentration in muscle could also be due to a reduced uptake of carnitine due to a decreased activity of OCTN, which, however, is unlikely with respect to the finding that OCTN in liver were up regulated in rats fed the oxidised fat. As muscle also has a high expression of PPARα, we expect that the expression of OCTN in muscle was increased rather than reduced by the dietary oxidised fat.
The present study further shows that a dietary oxidised fat leads to an up regulation of OCTN2 in the small intestine. As PPARα target genes (acyl-CoA oxidase, CYP4A1, CPT1a, CPT2) in the intestine were also up regulated in rats fed the oxidised fat, we assume that the increased expression of OCTN in intestine was also caused by activation of PPARα. As intestinal OCTN localised in the apical membrane of mucosa cells are able to transport carnitine from the diet into the cellReference Taylor27, Reference Kato, Sugiura, Sugiura, Wakayama, Kubo, Kobayashi, Sai, Tamai, Iseki and Tsuji28, an increased expression of these transporters may enhance their capacity to absorb carnitine. However, as ATB0+, another transporter involved in the intestinal absorption of carnitineReference Taylor27, was down regulated in rats fed the oxidised fat, it is difficult to draw conclusions about the whole intestinal absorption of carnitine from the diet. Nevertheless, the observed up regulation of intestinal OCTN may be relevant because they are polyspecific and do not only transport carnitine from the intestinal lumen into the mucosa cell but are also able to bind various drugs such as verapamil, spironolactone or mildronate and other monovalent cationsReference Wu, Huang, Prasad, Seth, Rajan, Leibach, Chen, Conway and Ganapathy14, Reference Kato, Sugiura, Sugiura, Wakayama, Kubo, Kobayashi, Sai, Tamai, Iseki and Tsuji28, Reference Koepsell and Endou55–Reference Hirano, Yasuda, Osaka, Kobayashi, Itagaki and Iseki58. As oxidised fats increase the gene expression of OCTN in the small intestine, it is possible that these fats also increase the absorption of various drugs from the intestine.
The hypothesis that the up regulation of OCTN was caused by PPARα activation provides also an explanation for the observed increased hepatic carnitine concentrations in fasted ratsReference McGarry, Robles-Valdes and Foster16, Reference Brass and Hoppel17. During fasting, NEFA are liberated from adipose tissue and act as activators of PPARα when they have entered the liver. Activation of PPARα up regulates many genes involved in hepatic mitochondrial and peroxisomal β-oxidation of fatty acids to supply acetyl-CoA used for the generation of ATP via the citrate cycle and for the generation of ketone bodies, an important fuel for the brain during fastingReference Kersten, Seydoux, Peters, Gonzalez, Desvergne and Wahli59, Reference Cheon, Nara, Band, Beever, Wallig and Nakamura60. These metabolic adaptations during fasting triggered by PPARα aim to minimise the use of protein and carbohydrates as fuel and allow mammals to survive long periods of energy deprivation. CPT are rate limiting for β-oxidation of fatty acidsReference Brandt, Djouadi and Kelly61, Reference Mascaro, Acosta, Ortiz, Marrero, Hegardt and Haro62. The up regulation of CPT, which is essential for the metabolic adaptations occurring during fasting, might increase the demand for carnitine in liver cells. We postulate that up regulation of OCTN by PPARα activation is a means to supply liver cells with sufficient carnitine required for the transport of excessive amounts of fatty acids into the mitochondrion, and therefore plays an important role in the adaptive response of liver metabolism to fasting.
In conclusion, the present study shows that an oxidised fat causes an up regulation of OCTN2 in the liver and small intestine of rats. As OCTN2 catalyses the uptake of carnitine into cells, these fats influence whole-body carnitine homeostasis. An increased hepatic carnitine concentration in rats treated with oxidised fat may be at least in part due to an increased uptake of carnitine from blood into the liver. Since OCTN2 binds not only carnitine but also various drugs, the possibility exists that increased OCTN2 expression in the small intestine may improve the absorption of various drugs.