Substitution of fishmeal (FM) and fish oil (FO) in aquafeeds by more readily available and sustainable alternative ingredients such as plant feedstuffs (PF) has become a priority issue in aquaculture( 1 ). However, dietary replacement of fishery by-products by PF may have negative health-related effects, especially in carnivorous species. For instance, vegetable oils (VO) were reported to induce alterations of the immune response in different fish species( Reference Montero, Kalinowski and Obach 2 – Reference Lin and Shiau 7 ), change the fatty acid profile of immune cells or modify eicosanoid production( Reference Montero, Kalinowski and Obach 2 , Reference Mourente, Good and Thompson 5 , Reference Bell, Strachan and Good 6 , Reference Ganga, Bell and Montero 8 ). Inflammation and pathological morphological modifications in the intestine and steatosis in the liver were also reported in several species fed VO-rich diets( Reference Olsen, Myklebust and Kaino 9 – Reference Kortner, Gu and Krogdahl 13 ). Inadequate dietary nutrient balance, high fibre or carbohydrate content and the presence of anti-nutritional factors in PF are possible causes for such effects( Reference Gatlin, Barrows and Brown 14 , Reference Montero and Izquierdo 15 ).
Oxidative stress occurs when balance between the generation of reactive oxygen species (ROS) exceeds that of ROS removal, thus inducing oxidative damage. ROS are produced at a controlled rate during normal cell metabolism( Reference Halliwell and Chirico 16 ). However, under stressful conditions such as nutritional alterations, ROS may dramatically increase and induce oxidative stress. Accordingly, variations in diet macronutrient sources( Reference Lin and Shiau 7 , Reference López-Bote, Diez and Corraze 17 – Reference Bañuelos-Vargas, López and Pérez-Jiménez 25 ), dietary protein:non-protein ratios( Reference Alvarez, López-Bote and Díez 26 – Reference Castro, Pérez-Jiménez and Guerreiro 29 ) or non-protein energy sources( Reference Wang, Liu and Lu 30 ) have been linked to modifications of fish susceptibility to lipid peroxidation (LPO).
The knowledge about the effects of macronutrients on oxidative stress is still limited and the available data are contradictory. Regarding the effect of dietary lipid source, VO were reported to either decrease( Reference Lin and Shiau 7 , Reference Menoyo, Izquierdo and Robaina 18 , Reference Peng, Chen and Qin 20 , Reference Gao, Koshio and Ishikawa 23 , Reference Ng, Chong and Wang 24 ), increase( Reference Ostbye, Kjaer and Rora 22 ) or promote no effects( Reference Kjaer, Aursnes and Berge 31 ) in fish hepatic and/or muscular LPO susceptibility. High dietary protein and/or lipid concentrations were observed to increase muscular LPO susceptibility( Reference Alvarez, López-Bote and Díez 26 , Reference Pérez-Jiménez, Hidalgo and Morales 28 ), whereas dietary carbohydrates decreased LPO in muscles and the liver( Reference Alvarez, López-Bote and Díez 27 , Reference Pérez-Jiménez, Hidalgo and Morales 28 , Reference Wang, Liu and Lu 30 ).
To prevent oxidative damage, organisms have evolved effective antioxidant systems, consisting of both non-enzymatic (GSH, vitamins and carotenoids, among others) and enzymatic mechanisms. Enzymes such as catalase (CAT), superoxide dismutase (SOD), glutathione peroxidase (GPX) and glutathione reductase (GR) play key roles in the antioxidant defence mechanism( Reference Mourente, Bell and Tocher 32 , Reference Lushchak 33 ). Available literature suggests that dietary lipid source has no effect on the liver and muscle activities of SOD, CAT and/or GPX( Reference Ostbye, Kjaer and Rora 22 , Reference Kjaer, Aursnes and Berge 31 , Reference Aminikhoei, Choi and Lee 34 ) or decreased SOD activity in VO-rich diets( Reference Kjaer, Todorcevic and Torstensen 35 ) in a number of fish species.
Concerning macronutrient modifications, it was reported that in common dentex (Dentex dentex) high dietary lipid concentrations induced an increase in hepatic SOD activity at high dietary protein concentrations, and of GPX, independent of dietary protein concentrations( Reference Pérez-Jiménez, Hidalgo and Morales 28 ). The authors also observed an interaction between dietary protein and carbohydrate or lipid concentrations in muscular GR and hepatic SOD and CAT activities. In yellow catfish (Pelteobagrus fulvidraco), a decrease in CAT and GPX activities as dietary carbohydrate:lipid ratio increased was also observed( Reference Wang, Liu and Lu 30 ). In contrast, partial substitution of dietary proteins with carbohydrates induced no major alterations in antioxidant enzyme activities in Senegalese sole (Solea senegalensis) liver( Reference Castro, Pérez-Jiménez and Guerreiro 29 ).
Overall, current knowledge about the effects of alternative feed ingredients, which may be rich in carbohydrates or VO, on the oxidative status of animals is still very scarce. Thus, in this study, we evaluated the effect of the dietary lipid source and carbohydrate manipulation on oxidative defence mechanisms in the liver and intestine of European sea bass (Dicentrarchus labrax) juveniles.
Methods
Experimental diets
Four diets differing in carbohydrate content (0 and 20 % gelatinised starch, CH– and CH+, respectively) and lipid source (fish oil (FO) or a VO blend, FO and VO, respectively) were formulated (Table 1). The dietary carbohydrate content was increased at the expense of dietary protein. The VO blend was composed of 20 % rapeseed, 50 % linseed and 30 % palm oils and replaced approximately 70 % of dietary lipids, which were provided by cod liver oil and FM. All the ingredients were finely ground, well-mixed and dry-extruded in a laboratory pellet mill (California Pellet Mill), through a 3-mm die. The pellets were air-dried for 24 h and stored in a refrigerator (4°C) until used.
Table 1 Ingredients and chemical composition of the experimental diets

FO, fish oil; VO, blend of vegetable oils; carbohydrate content, 0 % (CH–) or 20 % (CH+) gelatinised maize starch.
* Steam-dried low-temperature fishmeal; Pesquera Diamante (CP: 71·1 % DM; CL: 8·8 % DM).
† C-Gel Instant-12018; Cerestar.
‡ Labchem, Laborspirit Lda.
§ 30 % Palm oil (Colmi), 50 % linseed oil (Sociedade Portuense de Drogas) and 20 % rapeseed oil (Huilerie Emile Noël SAS).
|| Vitamins (mg/kg diet): retinyl acetate, 18 000 IU (6·19 mg)/kg diet; cholecalciferol, 2000 IU (0·04 mg)/kg diet; α-tocopherol acetate, 35; sodium menadione bisulphate, 10; thiamin-HCl, 15; riboflavin, 25; calcium pantothenate, 50; nicotinic acid, 200; pyridoxine HCl, 5; folic acid 10; cyanocobalamin, 0·02; biotin, 1·5; ascorbic acid, 50; inositol, 400 (Premix).
¶ Minerals (mg/kg diet): cobalt sulphate, 1·91; copper sulphate, 19·6; iron sulphate, 200; sodium fluoride, 2·21; potassium iodide, 0·78; magnesium oxide, 830; manganese oxide, 26; sodiumselenite, 0·66; zinc oxide, 37·5; dibasic calcium phosphate, 5·93 (g/kg diet); potassium chloride, 1·15 (g/kg diet); sodium chloride, 0·40 (g/kg diet) (Premix).
** Aquacube (Guar gum, polymethyl carbamide, Manioc starch blend, hydrate calcium sulphate) (Agil).
Animals, experimental conditions and sampling
This experiment was directed by accredited scientists (following FELASA category C recommendations) and conducted according to the European Union Directive (2010/63/EU) on the protection of animals for scientific purposes. The study was performed at the Marine Zoological Station, University of Porto, Portugal, in a thermo-regulated recirculation water system, equipped with twelve fibre-glass cylindrical tanks of 300 litre water capacity, and supplied with continuous flow of filtered seawater.
After 2 weeks of adaptation to the experimental conditions, twelve groups of twenty European sea bass juveniles (initial body weight 74·0 (sd 1·5) g) were established and randomly distributed into the tanks. The experimental diets were randomly assigned to triplicate groups of fish (n 3 tanks/diet). During the trial, salinity averaged 35 (sd 1·0) g/l, dissolved oxygen was kept near saturation and water temperature was regulated to 25·4 (sd 0·5)°C. The photoperiod was the natural one for June–August. The trial lasted 73 d, and during that period fish were hand-fed twice a day, 6 d/week, to apparent visual satiety. Food intake and mortality were recorded daily, and fish in each tank were bulk-weighted at the end of the trial, after 1 d of feed deprivation to empty gut contents. To eliminate handling stress, the fish were continued to be fed for one more week, and subsequently 18 h after the last meal (previous day afternoon meal) three fish per tank (n 9 fish/diet) were killed with a sharp blow to the head; the liver and intestines were removed, immediately frozen in liquid N2 and stored at –80°C until enzymatic analyses.
Diet analysis
Chemical analyses of the experimental diets were carried out according to Association of Official Analytical Chemists( 36 ) as follows: in brief, DM after drying at 105°C until constant weight; ash by incineration in a muffle furnace at 450°C for 16 h; protein content (N×6·25) by the Kjeldahl method after acid digestion using a Kjeltec digestion and distillation units (models 1015 and 1026, respectively; Tecator Systems); starch content according to Beutler( Reference Beutler 37 ); gross energy by direct combustion in an adiabatic bomb calorimeter (Parr model 6200; Parr Instruments); and lipid content by petroleum diethyl ether extraction (Soxtec HT System; Höganäs). Fatty acid methyl esters were prepared by acid-catalysed transmethylation of total lipids using boron trifluoride in methanol (14 %) according to Santha & Ackman( Reference Santha and Ackman 38 ) and were analysed by GC (Varian 3900; Varian) as described by Castro et al.( Reference Castro, Corraze and Panserat 39 ).
Enzymatic activity assays
Liver and whole intestine samples were homogenised (dilution 1:4) in ice-cold buffer (100 mm-Tris-HCl, 0·1 mm-EDTA and 0·1 % triton X-100 (v/v), pH 7·8). All the procedures were performed on ice. Homogenates were centrifuged at 30 000 g for 30 min at 4°C. After centrifugation, the resultant supernatant was collected, and the aliquots were stored at –80 °C until analysis.
All enzyme assays were carried out at 37°C. Changes in absorbance were monitored using a microplate reader (ELx808; BioTek Instruments) to determine enzyme activities. The optimal substrate and protein concentrations for maximal activity measurement of each enzyme were established by preliminary assays. The molar extinction coefficients used for H2O2 and NADPH were 0·039 and 6·22 mm −1×cm−1, respectively.
The specific assay conditions for each enzyme were as follows.
SOD (EC 1.15.1.1) activity was measured at 550 nm by the ferricytochrome C method using xanthine/xanthine oxidase as the source of superoxide radicals( Reference McCord and Fridovich 40 ). The reaction mixture consisted of 50 mm-potassium phosphate buffer (pH 7·8), 0·1 mm-EDTA, 0·1 mm-xanthine, 0·012 mm-cytochrome C and 0·025 IU/ml xanthine oxidase. Activity was reported as units per mg of protein. One unit of activity was defined as the amount of enzyme necessary to produce 50 % inhibition of ferricytochrome C reduction rate.
CAT (EC 1.11.1.6) activity was determined according to Aebi( Reference Aebi 41 ) by measuring the decrease in H2O2 concentration at 240 nm. The reaction mixture contained 50 mm-potassium phosphate buffer (pH 7) and 10 mm-H2O2 freshly added.
GPX (EC 1.11.1.9) activity was assayed as described by Flohé & Günzler( Reference Flohé and Günzler 42 ). The GSSG generated by GPX was reduced by GR, and NADPH consumption rate was monitored at 340 nm. The reaction mixture consisted of 50 mm-potassium phosphate buffer (pH 7·1), 1 mm-EDTA, 3·9 mm-GSH, 3·9 mm-sodium azide, 1 IU/ml GR, 0·2 mm-NADPH and 0·05 mm-H2O2.
GR (EC 1.6.4.2) activity was determined at 340 nm by measuring the oxidation of NADPH as described by Morales et al.( Reference Morales, Pérez-Jiménez and Hidalgo 43 ). The reaction mixture consisted of 0·1 m-sodium phosphate buffer (pH 7·5), 1 mm-EDTA, 0·63 mm-NADPH and 0·16 mm-GSSG.
Glucose-6-phosphate dehydrogenase (G6PD; EC 1.1.1.49) activity was assayed by measuring the reduction of NADP+ at 340 nm as previously described by Morales et al.( Reference Morales, García Rejón and de la Higuera 44 ), using a reaction mixture containing 50 mm-imidazole–HCl buffer (pH 7·4), 5 mm-MgCl2, 2 mm-NADP and 1 mm-glucose-6-phosphate.
Except for SOD, whose units of expression were described above, all other enzyme activities were expressed as units (CAT) or milliunits (G6PD, GPX and GR) per mg of soluble protein. One unit of enzyme activity was defined as the amount of enzyme required to transform 1 μmol of substrate/min under the above assay conditions. Protein concentration was determined according to Bradford( Reference Bradford 45 ) using a protein assay kit (Sigma) and bovine serum albumin as the standard.
Total glutathione, GSH and oxidative stress index
A portion of liver was homogenised in nine volumes of ice-cold solution containing 1·3 % 5-sulphosalicylic acid (w/v) and 10 mm-HCl. The procedure was carefully performed always on ice in order to avoid the GSH. Homogenates were centrifuged at 14 000 g for 10 min at 4°C, and the resulting supernatants were immediately analysed.
Total glutathione (tGSH) and GSSG were measured following the method described by Griffith( Reference Griffith 46 ) and Vandeputte et al.( Reference Vandeputte, Guizon and Genestie-Denis 47 ) with modifications. Both tGSH and GSSG analyses were carried out at 37°C, and the changes in absorbance as a consequence of the reduction of 5,5′-dithiobis (2-nitrobenzoic acid) (DTNB) were monitored at 405 nm using a microplate reader (ELx808; BioTek Instruments). The optimal substrate and protein concentrations for the measurement of maximal activity for each enzyme were established by preliminary assays. The molar extinction coefficient used for DTNB was 13·6 mm −1×cm−1.
tGSH was determined using a reaction mixture containing 133 mm-phosphate buffer with 5·8 mm-EDTA at pH 7·4, 0·71 mm-DTNB, 0·24 mm-NADPH and 1·2 IU/ml GR.
GSSG was measured using an aliquot from the solution obtained after 60 min of incubation of 100 μl of the sample with 2 μl vinylpyridine and 6 μl 1·5 m-triethanolamine. The reaction mixture contained 122 mm-phosphate buffer with 5·4 mm-EDTA at pH 7·4, 0·71 mm-DTNB, 0·24 mm-NADPH and 1·2 IU/ml GR.
The results were calculated using the standard curves of GSH and GSSG for tGSH and GSSG measurements, respectively. GSH level was calculated by subtracting GSSG from tGSH values. The results are expressed as nmol per g of tissue.
Oxidative stress index (OSI) was calculated as follows:
OSI (%)=100×(2×GSSG/tGSH).
Lipid peroxidation
Concentration of malondialdehyde (MDA) was determined as a marker for LPO following the methodology described by Buege & Aust( Reference Buege and Aust 48 ). An aliquot of supernatant from the homogenate (100 μl) was mixed with 500 μl of a previously prepared solution containing 15 % (w/v) TCA, 0·375 % (w/v) thiobarbituric acid (TBA), 80 % (v/v) HCl 0·25 n and 0·01 % (w/v) butylated hydroxytoluene. The mixture was heated to 100°C for 15 min. After being cooled to room temperature and centrifuged at 1500 g for 10 min, the absorbance was measured at 535 nm in the supernatant. MDA concentration was expressed as nmol MDA per g of tissue, calculated from a calibration curve.
Statistical analysis
Data are presented as mean values and standard deviations. Data were checked for normality and homogeneity of variances and when appropriate were normalised (log, inverse and arcsin
$\left( {\sqrt {\left( {x\,/\,{\rm 1}00} \right)} } \right)\ {\rm transformations})$
. Statistical evaluation of the data was carried out using a 2×2 factorial arrangement of treatments in a completely randomised experimental design (two-way ANOVA), with carbohydrate concentration and lipid source as fixed factors. The significance level of 0·05 was used for rejection of the null hypothesis. All the statistical analyses were carried out using SPSS 21.0 software package (IBM Corp.) for Windows.
Results
The diets presented small differences in the proportions of total SFA, which were slightly higher in the VO diets, and of MUFA, which were higher in the FO diets (Table 2). Within MUFA, higher concentrations of oleic acid (18 : 1n-9) were found in the VO diets, whereas the opposite occurred for palmitoleic acid (16 : 1n-7), eicosenoic acid (20 : 1n-9) and erucic acid (22 : 1n-9). Linoleic acid (LA, 18 : 2n-6), n-6 PUFA, was considerably higher in the VO diets. Regarding n-3 PUFA, VO diets were particularly rich in linolenic acid (LNA, 18 : 3n-3) and poor in EPA(20 : 5n-3) and DHA) (22 : 6n-3).
Table 2 Fatty acid composition (% of total fatty acids) of the experimental diets

FO, fish oil, VO, blend of vegetable oils; carbohydrate content, 0 % (CH–) or 20 % (CH+) gelatinised maize starch;·n-3 LC-PUFA, n-3 long-chain PUFA.
Results of the feeding trial were not the aim of this study and are presented elsewhere( Reference Castro, Corraze and Pérez-Jiménez 49 ).
In brief, growth performance and feed efficiency were not affected by dietary treatments. Final body mass averaged 221·7 (sd 5·0) g, feed efficiency (wet weight gain(g)/dry feed intake (g)) averaged 0·8 (sd 0·03) and feed intake (g/kg average body mass per d) averaged 16·6 (sd 0·7). Liver fatty acid composition is summarised in Table 3. Accordingly, liver samples of fish fed VO-based diets were characterised by higher concentrations of LA (18 : 2n-6), γ-LNA (GLA; 18 : 3n-6) and LNA (18 : 3n-3) and by lower concentration of arachidonic acid (ARA; 20 : 4n-6), EPA (20 : 5n-3) and DHA (22 : 6n-3) as well as n-3 PUFA:n-6 PUFA ratio. Except for ARA concentrations, lower in groups fed the CH+ diets, no other differences were found for hepatic n-6 PUFA concentrations. Regarding n-3 PUFA, groups fed the CH+ diets presented similar LNA concentrations but lower EPA and DHA concentrations compared with those fed CH– diets. Unsaturation index was lower in fish fed the CH+ diets. On the other hand, differences between lipid sources on unsaturation index were only evident in the CH– group. During the trial, no mortality occurred.
Table 3 Liver fatty acid (FA) profile (% of total FA) of European sea bass fed the experimental diets (Mean values and standard deviations; n 6 fish)

FO, fish oil; VO, blend of vegetable oils; CH content, 0 % (CH–) or 20 % (CH+) gelatinised maize starch.
* Significant differences at P<0·05 (two-way ANOVA).
Overall, both in the liver and in the intestines, there were no interactions between dietary carbohydrate and lipid source in the activities of oxidative stress enzymes or in LPO values. In the liver, dietary lipid source did not affect the activities of oxidative stress enzymes or LPO values, whereas dietary carbohydrates promoted a decrease in LPO values and in CAT activity and an increase in GR and G6PD activities (Table 4). Moreover, in the liver, SOD and GPX activities were not affected by dietary carbohydrates. In the intestine, the dietary carbohydrate level did not affect the activities of oxidative stress enzymes or LPO values, whereas dietary VO promoted a decrease in LPO and an increase in GPX and GR activities (Table 5). No effects on activities of CAT, SOD or G6PD in relation to diet composition were observed in the intestine.
Table 4 Specific activities of glucose-6-phosphate dehydrogenase (G6PD), glutathione peroxidase (GPX), glutathione reductase (GR) (mU/mg protein), catalase (CAT), superoxide dismutase (SOD) (U/mg protein) and lipid peroxidation (LPO) (nmol malondialdehyde per g tissue) in the liver of European sea bass fed the experimental diets(Mean values and standard deviations; n 9 fish)
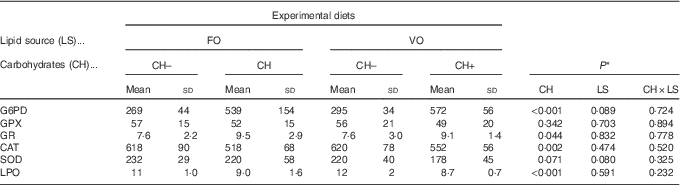
FO, fish oil;, VO, blend of vegetable oils; CH content, 0 % (CH–) or 20 % (CH+) gelatinised maize starch.
* Significant differences at P<0·05 (two-way ANOVA).
Table 5 Specific activities of glucose-6-phosphate dehydrogenase (G6PD), glutathione peroxidase (GPX), glutathione reductase (GR) (mU/mg protein), superoxide dismutase (SOD), catalase (CAT) (U/mg protein) and lipid peroxidation (LPO) (nmol malondialdehyde per g tissue) in the intestine of European sea bass fed the experimental diets(Mean values and standard deviations; n 9 fish)

FO, fish oil; VO, blend of vegetable oils; CH content, 0 % (CH–) or 20 % (CH+) gelatinised maize starch.
* Significant differences at P<0·05 (two-way ANOVA).
In the liver, higher GSH content and lower GSSG levels and OSI were observed in the carbohydrate groups (Fig. 1), but no effects of dietary lipid source on glutathione (total, reduced and oxidised) or OSI were observed.
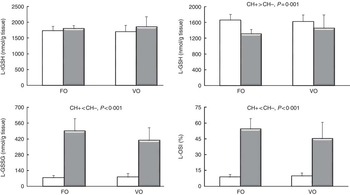
Fig. 1 Total glutathione (tGSH), GSH, GSSG and oxidative stress index (OSI) in the liver (L) of European sea bass fed experimental diets. Fish oil (FO), blend of vegetable oils (VO); carbohydrate content: 0 % (, CH–) or 20 % (
, CH+) gelatinised maize starch. Values are means (n 9 fish), with standard deviations represented by vertical bars. Significant differences at P<0·05 (two-way ANOVA).
Discussion
In this study, low susceptibility to LPO was observed in the liver of fish fed carbohydrate-rich diets, which is in agreement with previous observations on muscles of this and other fish species( Reference Alvarez, López-Bote and Díez 27 , Reference Pérez-Jiménez, Hidalgo and Morales 28 ). The beneficial effect of dietary carbohydrates on lipid oxidative damage may be related to the nature of the glucose molecule, which can scavenge ROS by itself( Reference Lygren and Hemre 50 ), or through increased activity of the pentose phosphate pathway( Reference Alvarez, López-Bote and Díez 27 , Reference Pérez-Jiménez, Hidalgo and Morales 28 , Reference Lygren and Hemre 50 , Reference Sagone, Greenwald and Kraut 51 ). The pentose phosphate pathway, via G6PD, generates reducing power in the form of NADPH, which is required for the activity of two H2O2-scavenging pathways involving CAT and GPX( Reference Morales, Pérez-Jiménez and Hidalgo 43 , Reference Nelson and Cox 52 ). In fact, along with lower hepatic LPO levels, higher G6PD activity was observed in European sea bass fed carbohydrate-rich diets. Furthermore, dietary carbohydrates also promoted an increase in hepatic GR activity, an enzyme that also requires NADPH to regenerate reduced glutathione (GSH) to its oxidised form (GSSG). Consistent with this observation, higher concentration of GSH and lower concentration of GSSG were observed in the liver of fish fed carbohydrate-rich diets. GSH is an essential substrate for GPX, but as it is also an effective ROS scavenger it plays an important role by preventing some of the deleterious effects of oxidative stress( Reference Wu, Fang and Yang 53 ). Accordingly, liver OSI, a biomarker of the cellular redox state, was also lower in groups fed carbohydrate-rich diets. Studies using OSI to assess the effects of oxidative stress in fish are scarce( Reference Olsvik, Kristensen and Waagbo 54 – Reference Pérez-Jiménez, Peres and Cruz Rubio 58 ) and mainly report a huge variation in OSI values (from 0·6 to 30 %) at the hepatic level( Reference Olsvik, Kristensen and Waagbo 55 – Reference Pérez-Jiménez, Peres and Cruz Rubio 58 ). As several variables (such as fish species, abiotic factors, fish size, time elapsed from the last meal or nutritional history) may regulate OSI values, comparisons among studies or establishment of reliable reference values for this parameter are difficult. Furthermore, a number of parameters or techniques have traditionally been used as measurements of oxidative stress. Among the most studied are antioxidant defence enzymes, TBA reactive substances (TBARS), concentrations of tGSH and GSSG and resulting OSI. In this study, the response of most parameters analysed (including OSI) was well correlated. However, other studies( Reference Olsvik, Kristensen and Waagbo 55 , Reference Pérez-Jiménez, Peres and Cruz Rubio 58 ) have demonstrated that OSI was not a suitable biomarker of oxidative stress under stressful conditions. As stated by Peres et al.( Reference Peres, Santos and Oliva-Teles 59 ), reliable parameters are those that respond to stressful conditions but have low variation under normal conditions. This highlights the importance of taking into consideration the response of more than one parameter involved in the oxidative status( Reference Olsvik, Kristensen and Waagbo 55 , Reference Pérez-Jiménez, Peres and Cruz Rubio 58 ).
On the other hand, the carbohydrate-rich diets promoted a lower hepatic CAT activity. Previously, Lygren & Hemre( Reference Lygren and Hemre 50 ) also reported that hepatic CAT and SOD activities decreased in Atlantic salmon (Salmo salar) fed high-carbohydrate diets. These observations suggest that the lower LPO observed in fish fed the carbohydrate diets was mainly related with the higher concentrations of reducing equivalents such as NADPH and GSH and that activation of primary antioxidant enzymes, which are more energy-demanding, was not required.
Fatty acid composition of reserve lipids in tissues (liver, muscle, intestine, etc.) reflects dietary fatty acid profile( Reference Rosenlund, Corraze and Izquierdo 60 ). In the present study, the reduced n-3:n-6 ratios (2·3 to 2·7 in VO groups v. 7·5 to 5·4 in FO groups) in the hepatic fatty acid profile of European sea bass fed VO-blend diets are consistent with previously reported values (1·9 to 2·5 in VO groups v. 4·3 in FO group) for this species also fed similar VO-blend based diets( Reference Mourente, Good and Thompson 5 ). FO is rich in long-chain PUFA (LC-PUFA), whereas VO is rich in PUFA, and it is well established that due to their high degree of unsaturation LC-PUFA are more susceptible to peroxidation than PUFA or MUFA, thus promoting the formation of more free radicals( Reference Halliwell and Chirico 16 ). Accordingly, in this study, higher hepatic LC-PUFA content was observed in European sea bass fed the FO-based diets than the VO-rich diets( Reference Castro, Corraze and Pérez-Jiménez 49 ), although liver oxidative status was not affected by dietary lipid source. In fact, although unsaturation index was lower in fish fed the carbohydrate-rich diets, differences related to lipid sources were only evident in the CH– group (lower in VO diet). Similarly, partial replacement of FO by VO did not induce changes in hepatic lipid oxidative damages and antioxidant enzyme activities of Atlantic cod (Gadus morhua) or rockfish (Sebastes schlegeli)( Reference Kjaer, Aursnes and Berge 31 , Reference Aminikhoei, Choi and Lee 34 ) or in LPO concentration in Japanese sea bass (Lateolabrax japonicas)( Reference Gao, Koshio and Ishikawa 23 ).
In contrast to what was observed in the liver, in the present study, the dietary lipid source affected the intestinal oxidative status in European sea bass juveniles. Overall, oxidative lipid stress measured as LPO concentration was higher in fish fed the FO-based diets than the VO-rich diets. Studies addressing the effect of dietary lipid source on fish oxidative status were mainly focused on liver and muscle tissues( Reference Lin and Shiau 7 , Reference Menoyo, Izquierdo and Robaina 18 , Reference Peng, Chen and Qin 20 , Reference Ostbye, Kjaer and Rora 22 – Reference Ng, Chong and Wang 24 , Reference Kjaer, Aursnes and Berge 31 , Reference Kjaer, Todorcevic and Torstensen 35 ). To the best of the authors’ knowledge, the impact of dietary lipid source on intestinal oxidative status of fish was only evaluated in Japanese sea bass, and it was limited to the assessment of LPO concentration( Reference Gao, Koshio and Ishikawa 23 ). In that study, dietary inclusion of palm oil led to decreased intestinal LPO concentration. Available studies dealing with the modulator role of nutrition on intestinal antioxidant enzymatic mechanisms were mainly dedicated to the role of amino acids( Reference Wen, Feng and Jiang 61 , Reference Zhao, Hu and Zhou 62 ), anti-nutritional factors( Reference Zhang, Guo and Feng 63 ) and NSP( Reference Enes, Pérez-Jiménez and Peres 64 ).
GPX catalyses the reduction of H2O2 and lipid peroxides (ROOH) into the corresponding stable alcohols (ROH) at the expense of GSH( Reference Mourente, Bell and Tocher 32 ). GR catalyses the reduction of GSSG to GSH and its activity is tightly bound to that of GPX, in an attempt to keep the adequate GSSG:GSH ratio necessary for GPX function. In the present study, GPX and GR activities were responsive to dietary treatments and had increased activities in groups fed the VO diets. Although flavonoids and phenolic compounds of VO were not measured in the present study, their potential role in the intestinal GPX and GR activation cannot be discarded. There is evidence that some plant constituents (flavonoids, phenolic compounds) also present in VO have antioxidant functions in mammals( Reference Nagata, Takekoshi and Takagi 65 – Reference Procházková, Boušová and Wilhelmová 67 ). This antioxidant effect may result from a direct free-radical scavenging action by these compounds or from their role in activation of important antioxidant enzymes( Reference Nagata, Takekoshi and Takagi 65 – Reference Procházková, Boušová and Wilhelmová 67 ). Accordingly, Nagata et al ( Reference Nagata, Takekoshi and Takagi 65 ) observed in vitro that in rat hepatocytes two flavonoids (quercetin and catechin) exert their antioxidant activity against H2O2 through activation of GPX. In human hepatocytes, it was also demonstrated that cocoa flavonoids exert an antioxidant protective effect against tert-butyl hydroperoxide via induction of GPX and GR activities. In fish, studies are not yet available. However, Sitjà-Bobadilla et al.( Reference Sitjà-Bobadilla, Peña-Llopis and Gómez-Requeni 68 ) suggested that replacement of FM by a mixture of plant ingredients in diets for gilthead sea bream (Sparus aurata) exerts an antioxidative effect through activation of glutathione metabolism, as plant-containing diets increased muscular GSH and hepatic glutathione redox status, as well as GR activity, in both hepatic and muscular tissues. According to the authors, this increased antioxidant potential related to plant ingredients was probably linked to the presence of phenolic compounds and/or flavonoids. Further, Saera-Vila et al.( Reference Saera-Vila, Benedito-Palos and Sitjà-Bobadilla 69 ) reported that FO replacement by VO in plant protein-based diets increased hepatic GSH:GSSG ratio, despite the reduction of absolute glutathione concentrations. According to the authors, this suggests that fish fed these diets may be less susceptible to LPO and oxidative damage. Overall, both the fatty acid profile of VO-based diets and the presence of plants constituents with antioxidant potential may explain the lower intestinal susceptibility to oxidative stress/damages in VO groups.
Transcriptional and proteomic studies have also evidenced that intestinal oxidative status may be affected by changes in dietary lipid source( Reference Olsvik, Torstensen and Berntssen 70 , Reference Morais, Silva and Carneiro 71 ). Accordingly, Morais et al. ( Reference Morais, Silva and Carneiro 71 ) reported that in Atlantic salmon the dietary FO substitution by VO led to an up-regulation of transcripts and proteins involved in detoxification and protection from oxidative stress, CAT and selenoprotein, as well as haemopexin-like protein and peroxiredoxin-1 proteins. On the contrary, a previous study on the same species showed that dietary FO replacement by VO decreased the transcription levels of antioxidant-related genes such as glutathione S-transferase and GR( Reference Olsvik, Torstensen and Berntssen 70 ).
Similarly to our previous studies on sturgeon (Acipenser naccarii) and rainbow trout (Oncorhynchus mykiss)( Reference Trenzado, Hidalgo and García-Gallego 72 ), in this study, European sea bass intestines also showed LPO damage values and GPX activity 2- to 5-fold higher compared with the liver. GPX and GR activities were also 2-fold higher in the intestine than in the liver of European sea bass. On the contrary, G6PD and CAT activities were, respectively, more than 10- and 5-fold lower in the intestine than in the liver. These differences between the two organs are a reflex of the distinct antioxidant mechanism strategies that are more relevant in each organ. The intestine being a tissue with high cell turnover is highly susceptible to oxidative status, and seems to require high activity of antioxidant enzymes.
In conclusion, results of the present study indicate that liver and intestine oxidative status balance were regulated through different metabolic pathways, and that dietary macronutrients affected metabolic pathways differently in each tissue. In addition, the observed LPO values suggest that susceptibility to oxidative stress was more important in the intestine than in the liver. Dietary carbohydrates contributed to reduced oxidative stress only in the liver, whereas the intestine was exclusively responsive to the dietary lipid source, with VO having a positive effect on the reduction of oxidative stress. Nowadays a wide range of PF are already used in commercial aquafeeds, including cereals and peas, which are rich sources of starch, and may therefore have a protective effect on liver oxidative stress. Given the importance of the intestine on whole-body homoeostasis and health, increased attention should be paid to this tissue in future studies evaluating the impact of dietary alternative ingredients on oxidative stress.
Acknowledgements
The authors express their thanks to P. Correia for technical assistance and L. Larroquet for the fatty acid analyses.
This work was partially supported by the FCT (Foundation for Science and Technology), Portugal (project PTDC/MAR-BIO/4107/2012), and co-financed by the European Regional Development Fund (ERDF) through the COMPETE – Operational Competitiveness Programme and national funds through FCT – under the project ‘PEst-C/MAR/LA0015/2011’. C. C., A. P.-J. and F. C. were supported by grants (SFRH/BD/76297/2011; SFRH/BPD/64684/2009; SFRH/BD/86799/2012, respectively) from FCT. P. D.-R. and C. R. S. were supported by grants (NORTE-07-0124-FEDER-000038-BPD-2013-07; NORTE-07-0124-FEDER-000038-BPD-2013-05, respectively).
C. C. carried out the main experimental work and wrote the draft of the manuscript under the direction of the project designer and leader A. O.-T., G. C., S. P. A. P.-J., F. C., P.D.-R., C. R. S. and H. P. assisted with the biochemical analyses and draft writing. All the authors contributed to and approved the manuscript.
The authors declare that there are no conflicts of interest.