Food oral processing has been extensively studied for its contribution to sensory perception and food intake. Recent studies explored the impact of food oral processing on subsequent nutrient digestion. Oral processing behaviour influences bolus properties, nutrient release and utilisation during digestion(Reference Mioche, Bourdiol and Peyron1). Individual differences between consumers in oral processing behaviour can contribute to the formation of boli differing in properties. Bolus properties change with oral processing time and are product specific. For foods such as gels, meats, cheeses and sausages, an increase in the number of bolus particles and a decrease in size of bolus particles were observed with prolonged chewing time(Reference Devezeaux de Lavergne, van Delft and van de Velde2,Reference Mosca, van de Velde and Bult3) .
The degree of food breakdown during oral processing can influence nutrient digestion and utilisation. An in vivo study observed that mastication enhanced accessibility for amylases to starch granules by damaging cellular structures(Reference Zhou, Topping and Morell4). In subjects with normal glucose tolerance, thorough mastication (chewing time = 30 s) increased early-phase insulin secretion and effectively reduced post-prandial plasma glucose concentrations after mastication of a meal of hamburger and rice compared with usual mastication (chewing time = 10 s), indicating a faster digestion of carbohydrates with longer chewing time(Reference Suzuki, Fukushima and Okamoto5). However, in subjects pre-disposed to type 2 diabetes, thorough mastication did not affect early-phase insulin secretion compared with usual mastication. These findings suggest that the effect of oral processing behaviour on metabolic response and macronutrient utilisation may differ between individuals and between populations. An inverse correlation between bolus particle size and glycaemic response was observed for rice (chewing time ranged from 18 to 27 s between subjects) indicating that inter-individual differences in mastication behaviour may cause inter-individual differences in starch digestion of rice. However, in the same study, this inverse correlation between bolus particle size and glycaemic response was not observed for spaghetti (chewing time ranged from 17 to 31 s between subjects)(Reference Ranawana Viren, Henry and Pratt Megan6). Studies with almonds(Reference Grundy, Grassby and Mandalari7–Reference Mandalari, Parker and Grundy9), pistachios(Reference Chessa, Filocamo and Faulks10) and hazelnuts(Reference Capuano, Pellegrini and Ntone11) revealed that mastication or mechanical breakdown of the nut matrix ruptures cell walls thereby increasing in vitro or in vivo lipid digestion compared with the intact nut matrix. This suggests that the degree of mastication influences the amount of lipids released from nuts. Long chewing (forty chews) of almonds led to higher in vivo lipid absorption (lower faecal fat excretion) than short chewing (ten chews)(Reference Cassady, Hollis and Fulford12). An in vivo study of walnuts, almonds and pistachios suggested that differences in bolus particle size at swallowing can lead to differences in energy release from nuts(Reference Capuano, Oliviero and Fogliano13). As a consequence, actual bio-accessibility of nutrients as well as actual energy content of nuts after consumption may be less than theoretically predicted(Reference Capuano, Oliviero and Fogliano13). To summarise, mechanical breakdown of foods during oral processing can contribute to the accessibility of food bolus fragments to digestive enzymes, leading to an increase in digestion efficiency and alteration of the kinetics of gastrointestinal utilisation of nutrients. Currently, a detailed understanding of the contribution of inter-individual differences in food oral processing behaviour of consumers on inter-individual differences in macronutrient digestion, metabolic responses and macronutrient utilisation is lacking.
Surprisingly, the effect of oral processing behaviour on protein digestion has been hardly studied, while proteins are an essential macronutrient playing numerous structural and functional roles in foods and contributing to health. One study compared elderly subjects of denture wearers with subjects with healthy, natural dentition and reported that meat protein utilisation was impaired by insufficient chewing(Reference Rémond, Machebeuf and Yven14). Minced beef was more rapidly digested and absorbed by elderly subjects than beef steak, which indicates that smaller food particles lead to higher nutrient availability(Reference Pennings, Groen and Van Dijk15). These studies highlighted that the higher degree of food degradation at the swallowing moment can enhance protein digestion and utilisation in the human body. However, these studies do not allow to determine whether naturally occurring difference in oral processing behaviour of consumers is sufficient to cause difference in subsequent protein digestion.
The aim of the present study was to determine the influence of naturally occurring oral processing behaviour on bolus properties and in vitro protein digestion of chicken and a soya-based vegetarian equivalent. Chicken and its soya-based equivalent were chosen as foods because of the growing global interest in plant-based meat analogues. Two chewing times corresponding to naturally occurring short and long mastication were chosen for both foods. Expectorated boli chewed by healthy adults were subjected to in vitro gastric and intestinal protein digestion. The use of a highly standardised in vitro digestion method allowed to exclude inter-individual differences in protein digestion caused by other factors than oral processing behaviour. We hypothesise that long chewing time leads to the formation of more and smaller bolus fragments with increased surface area which increases gastric and intestinal in vitro protein digestion compared with short chewing time.
Materials and methods
Materials
Chicken strips (AH Fried chicken filet slices Caesar, Albert Heijn BV; hereafter referred to as chicken (C)), soya-based vegetarian chicken strips (Vegan NOCHICKEN Chunks, a soya-based vegetarian chicken equivalent, The Vegetarian Butcher; hereafter referred to as vegetarian chicken (V)) and sunflower oil (Jumbo Sunflower oil, Jumbo Supermarkten BV) were purchased from local supermarkets.
Pepsin (P6887, 3200–4500 units/mg protein), pancreatin (P1750, 4 × USP specification), porcine bile extract (B8631), Pefabloc® SC, sodium dodecyl sulphate, o-phthaldialdehyde, dl-dithiothreitol and l-serine were purchased from Sigma-Aldrich Ltd. Trichloroacetic acid (TCA) (CAS 76-03-9) and disodium tetraborate decahydrate (Borax, CAS 1303-96-4) were bought from Merck & Co. All other chemicals used in the present study were of analytical grade. Enzymes used in simulated digestive fluids were assayed for their activity and diluted to reach the required activity based on the INFOGEST 2.0 protocol(Reference Brodkorb, Egger and Alminger16).
Preparation of chicken and vegetarian chicken
Commercially available chicken (C) and soya-based vegetarian chicken (V) were cut into piece of around 5 g. Chicken was chicken breast meat which was sliced, cooked and seasoned by the producer. Chicken was used without further preparation. Vegetarian chicken was stir fried on both sides in around 5 g of sunflower oil (brushed evenly on the pan) at medium heat for around 5 min until crispy. According to the nutritional label on the package, chicken consisted of 94 % chicken fillet (94 g/100 g), natural flavouring, salt (1·2 g/100 g), dextrose (0·4 g/100 g), dietary fibre (citrus, bamboo, potato), potato starch, chicken protein, vinegar and spices. According to the nutritional label on the package, vegetarian chicken consisted of 93 % soya structure (water, soya protein concentrate, salt), sunflower oil and natural flavouring. The protein content determined using DUMAS (see the Determination of protein content section) was 31·9 ± 1·1 % for chicken and 23·0 ± 1·1 % for soya-based vegetarian chicken.
Determination of natural chewing time of chicken and vegetarian chicken
Natural chewing time of chicken and vegetarian chicken
Natural chewing time of chicken and vegetarian chicken was determined in n 96 participants (fifty-seven females and thirty nine males; aged 25 ± 6 years). Sex, age, nationality, educational level, living time in the Netherlands, body weight and height, allergies and intolerances, dental status and smoking condition were collected from all participants using questionnaires. Chewing time was determined by providing 5 g of chicken or vegetarian chicken (one piece) to the participants. Participants were instructed to chew naturally on the piece and to raise their hand to indicate the moment of swallowing. Participants were video recorded during consumption. Chewing time (s) was extracted from the videos as the time period between placing the food into the mouth until swallowing. Number of chews was extracted from the videos to obtain chewing frequency (per s). All participants gave written informed consent. All participants received financial reimbursement for their participation in the study. The study does not fall within the remit of the ‘Medical Research Involving Human Subjects Act’. All work has been done in agreement with the WMA Declaration of Helsinki about Ethical Principles for Medical Research Involving Human Subjects.
K-means cluster analysis of chewing time
K-means cluster analysis was performed on chewing time (n 96) to determine clusters of subjects differing in natural chewing time to select shortest and longest chewing times. IBM SPSS Statistics (version 25.0) was used. To obtain representative clusters for naturally occurring short and long chewing time, we considered that clusters should represent at least 15 % of the population (n ≥ 15) and that clusters should be comparable in size. Clusters with less than two subjects (n < 2) were considered outliers. The selected two clusters represent the shortest and the longest chewing time as they occur when participants naturally chewed both foods and represent at least 30 % of the total population.
Collection of chicken and vegetarian chicken boli
Expectorated boli of chicken and vegetarian chicken chewed for 11 and 24 s (see the Natural oral processing behaviour of chicken and soya-based vegetarian chicken section) were collected from sixteen participants (nine females and seven males, eight Chinese and eight Dutch; aged 26 ± 8 years). Bolus properties were characterised immediately after expectoration (see the Characterisation of bolus properties section). To determine in vitro gastric and intestinal protein digestion, expectorated boli were collected from seven participants (four females and three males, seven Chinese; aged 24 ± 1 years). All participants were asked to chew 5 g of chicken or soya-based vegetarian chicken by following a referential instruction video. The instruction video showed a person chewing chicken for 11 or 24 s with a chewing frequency of 1·4 chews/s. The instruction video also provided a prompt tone every 0·7 s when a chew was taken. Participants watched the video with the prompt tones while masticating and were instructed to mimic the chewing behaviour shown in the video. Food boli after mastication were expectorated into containers and labelled as C11 (chicken chewed for 11 s), C24 (chicken chewed for 24 s), V11 (vegetarian chicken chewed for 11 s) and V24 (vegetarian chicken chewed for 24 s), respectively.
Characterisation of bolus properties
Particle number and particle size of bolus fragments were determined by image analysis(Reference Van Eck, Wijne and Fogliano17). In brief, 0·5 g of expectorated bolus was placed in a Petri dish (120 × 120 × 17 mm). All measurements were done in duplicate directly after expectoration. Individual bolus fragments were gently separated manually using a spatula. Petri dishes were placed on a flatbed scanner (Canon CanoScan 9000 F MarkII), and a 600-dpi colour picture was taken with a black background. Pictures were imported into ImageJ (version 1.52a, National Institute of Health) to conduct image analysis. Pictures were converted to an eight-bit image, after which a brightness/contrast adjustment and a black and white threshold were applied to obtain a binary image. For each image, the number of bolus particles and bolus particle mean area (mm2) as a measure of bolus particle size were obtained and standardised per g bolus(Reference Van Eck, Wijne and Fogliano17). Particles smaller than 0·07 mm2 or with a circularity <0·15 were discarded from data analysis to prevent interference of background. In a preliminary study, we found that typically 3–8 wt% of chicken and soya-based vegetarian chicken particles in the expectorated bolus were smaller than 0·07 mm2. We acknowledge that bolus particle number is slightly underestimated and bolus particle size is slightly overestimated with this experimental protocol.
Determination of protein content
Protein content of chicken and vegetarian chicken was determined in triplicate after drying at 60°C for 24 h using the DUMAS method with a Flash EA 1112 NC analyser (Thermo Fisher Scientific Inc.) following the manufacturer’s protocol. Protein content was calculated with a conversion factor of 6·25. Total protein content of food bolus was determined in triplicate using the same method.
In vitro gastric and intestinal protein digestion
In vitro gastric protein digestion
An independent set of expectorated boli were collected from n 16 participants (see the Collection of chicken and vegetarian chicken boli section) after different chewing time (11 and 24 s) for chicken and vegetarian chicken. Boli were subjected to in vitro gastric protein digestion immediately after expectoration. In vitro digestion for the determination of protein hydrolysis was performed for each bolus according to the harmonised INFOGEST 2.0 protocol(Reference Brodkorb, Egger and Alminger16). First, the food bolus was weighed and diluted 1:1 w/w with Milli-Q water to reach the same volume as the in vitro oral digesta described in INFOGEST 2·0, e.g. 5·1 g bolus+5·1 ml Milli-Q water. Second, the mixture was added to pre-warmed simulated gastric fluid (SGF) and pepsin (2000 U/ml final concentration). The pH was adjusted to 3 with 5 m HCl before 2 h incubation at 37°C to simulate the gastric phase. In vitro digestion was performed in a laboratory incubator with constant rotation by rotator. Chyme aliquot (0·5 ml) was taken after digested for 0, 30, 60 and 120 min, and 12·5 µl of 5 m NaOH was added to stop gastric digestion. Blank gastric digestion was determined by replacing boli sample by 5 g of Milli-Q water.
In vitro intestinal protein digestion
An independent set of expectorated boli were collected from n 7 participants (see the Collection of chicken and vegetarian chicken boli section) after different chewing time (11 and 24 s) for chicken and vegetarian chicken. Boli were subjected to in vitro intestinal protein digestion. First, oral pre-treatment and in vitro gastric digestion were done as described in the In vitro gastric protein digestion section. For intestinal digestion, gastric chyme was combined with pre-warmed simulated intestinal fluid (SIF), pancreatin (100 U/ml for trypsin in pancreatin final concentration) and bile salts (10 mm final concentration). The pH was raised to 7 with 5 m NaOH before incubation at 37°C for another 2 h. In vitro digestion was performed in a laboratory incubator under constant rotation by rotator. Four chyme aliquots (0·5 ml/aliquot) were taken during the gastric digestion, and two chyme aliquots were taken during the intestinal digestion (60 and 120 min). The values for the end points of gastric digestion at 120 min correspond to the values of the intestinal digestion at 0 min. To stop intestinal digestion, 2·5 µl of 0·1 m protease inhibitor Pefabloc® SC (5 mm final concentration) was added in each intestinal aliquot. Blank digestion for intestinal phase were performed by replacing boli samples by 5 g of Milli-Q water.
Separation of free amino acids and peptides
Free amino acids and peptides from the in vitro digested samples were separated by precipitating intact and undigested protein by TCA addition(Reference Mulet-Cabero, Rigby and Brodkorb18). The mixture of 0·25 ml of the digested sample and 0·415 ml of 5 % TCA was centrifuged at 10 000 g at 20°C for 30 min. Supernatant was filtered using a 0·45 μm syringe filter (15 mm diameter, 0·45 μm pore size polyvinylidene difluoride (PVDF) membrane)(Reference Zahir, Fogliano and Capuano19). The filtrate was stored at −20°C until further analysis.
Determination of protein hydrolysis
Free amino groups released after in vitro digestion were quantified using the o-phthaldialdehyde spectrophotometric assay according to the method described by Nielsen et al. (Reference Nielsen, Petersen and Dambmann20). Total content of free amino groups of chicken and vegetarian chicken was obtained from unchewed foods by acid hydrolysis in 6 m HCl at 110°C for 24 h. o-Phthaldialdehyde reagent was freshly prepared every time before measurements and used within 2 h. A quantity of 1 μl of fully hydrolysed unchewed sample was diluted 400× by adding 399 μl phosphate buffer (pH 7) to obtain 400 μl, while 8 μl digested samples were diluted 50× by adding 392 μl phosphate buffer (pH 7) to obtain 400 μl before o-phthaldialdehyde measurements. Free amino group concentration of in vitro digested and fully hydrolysed unchewed samples was determined with reference to a calibration curve by using l-serine (6·25–100 mg/l) standard solution prepared in phosphate buffer (pH 7). Three ml of o-phthaldialdehyde reagent was added to 400 μl of each sample, including standard solution, blank digestion and digested sample. The mixture was mixed for 5 s by vortex then stood at room temperature for 2 min. Absorbance was measured at 340 nm. Absorbance of blank digestion (Milli-Q water replacing food bolus, at time 0 min of the gastric phase and time 0 min of the intestinal phase) was subtracted from all absorbances to eliminate the interference of digestive solutions.
The value of NH2 content was normalised by protein content of each corresponding sample which was determined by DUMAS (see the Determination of protein content section) due to the different weight and protein content of bolus samples. The degree of protein hydrolysis (DH%) was calculated as

with:
NH2 (digestion) = free amino groups in hydrolysate of digested sample
NH2 (initial) = free amino groups in undigested sample (at time = 0 min of gastric or intestinal digestion)
NH2 (acid) = total content of free amino groups in unchewed sample fully hydrolysed in 6 m HCl at 110°C for 24 h
m(digestion) = weight of protein in food bolus before in vitro digestion
m(acid) = weight of protein in food matrix before acid hydrolysis.
Statistical data analysis
To investigate the influence of chewing time and food type on bolus properties, linear mixed models were used with food type (chicken and vegetarian chicken), chewing time and sex as fixed factors and nationality and subject as random effects.
To investigate the influence of chewing time and food type on in vitro protein digestion and to validate the compliance to the video instruction, linear mixed models were used with food type, chewing time, digestion time, sex and the interaction of food matrix × chewing time as fixed effects and nationality and subject as random effects on in vitro gastric digestion, while the effects of sex and nationality were excluded in in vitro intestinal protein digestion.
Paired t tests were used to compare in vitro protein hydrolysis between chewing time of 11 and 24 s at the end of the simulated gastric phase and at the end of the simulated intestinal phase for both foods.
Bivariate Pearson correlation (two-tailed) tests were used to examine the relationships between bolus properties (number, mean area) and in vitro protein gastric and intestinal hydrolysis for each of the two foods.
IBM SPSS Statistics (version 25.0) was used to perform all statistical analysis. A significance level of P < 0·05 was chosen.
Results
Natural oral processing behaviour of chicken and soya-based vegetarian chicken
Table 1 shows the results of the cluster analysis of the natural chewing time of chicken and vegetarian chicken as well as the mean values of all clusters.
Table 1. Results of cluster analysis of chewing time (s) of chicken and vegetarian chicken (n 96)*
(Mean values and standard deviations)

* Consumers were clustered in five groups indicated as a, b, c, d and e. Percentages represent the proportion of participants in a cluster relative to all participants (n 96).
† Groups representative for short and long chewing times.
In the clustering of natural chewing time of chicken, twenty-four out of ninety-six participants (25·0 %) were grouped as short eaters of chicken (short chewing time 10·9 (sd 1·7) s), and twenty-nine out of ninety-six participants (30·2 %) were grouped as long eaters (long chewing time 22·5 (sd 2·5) s) of chicken. Therefore, the selected short and long chewing time groups for chicken represent 55·2 % of all participants. In the clustering of natural chewing time of vegetarian chicken, eighteen out of ninety-six participants (18·8 %) were grouped as short eaters of vegetarian chicken (short chewing time 10·7 (sd 1·7) s), and sixteen out of ninety-six (16·7 %) participants were grouped as long eaters of vegetarian chicken (long chewing time 25·2 (sd 2·5) s). The selected short and long chewing time groups for vegetarian chicken represent 35·5 % of all participants. Natural chewing time of short eaters of chicken (10·9 (sd 1·7) s) is similar to short eaters of vegetarian chicken (10·7 (sd 1·7) s). The same is true for long eaters of chicken (22·5 (sd 2·5) s) and vegetarian chicken (25·2 (sd 2·5) s). Short eaters consumed chicken with a slightly higher (P = 0·015) chewing frequency (1·5 (sd 0·4) chews/s) than long eaters (1·3 (sd 0·2) chews/s). No significant differences in chewing frequency of vegetarian chicken were found between short eaters (1·4 (sd 0·3) chews/s) and long eaters (1·3 (sd 0·2) chews/s). To summarise, chewing time was roughly twice as long for long chewers compared with short chewers while chewing frequency remained similar between groups. The similarity of natural chewing time in these two matrices made it possible to use the same instruction videos for chewing of both matrices. The average of two short chewing times (10·8 s ≈ 11 s) and two long chewing times (23·8 s ≈ 24 s) were used as instructions for short and long chewing for chicken and vegetarian chicken to obtain the boli. An average chewing frequency of 1·4 chews/s was used for all chewing time and both foods.
Influence of chewing time on bolus properties of chicken and vegetarian chicken
In Table 2, representative images of expectorated boli and separated boli particles as well as bolus properties (number and mean area of bolus particles) are shown.
Table 2. Bolus properties of chicken and soya protein-based vegetarian chicken chewed for 11 and 24 s: (A) representative pictures of expectorated boli of one subject; (B) representative scans of separated boli particles of one subject; (C) number of bolus particles; (D) mean area of bolus particles*
(Mean values and standard deviations for (C) and (D); n 16; duplicate)

C11, chicken chewed for 11 s (short chewing time); C24, chicken chewed for 24 s (long chewing time); V11, vegetarian chicken chewed for 11 s (short chewing time); V24, vegetarian chicken chewed for 24 s (long chewing time).
a,b,c,d Unlike letters indicate significant differences between means of short and long chewing times (P < 0·001).
* Number and mean area of bolus particles were normalised by the weight of scanned bolus.
For both foods, a long chewing time (24 s) resulted in formation of significantly (P < 0·001) more and smaller bolus fragments than a short chewing time (11 s), which confirms our hypothesis (see the Introduction section). Long chewing (24 s) of chicken generated 6612 (sd 1587) fragments per g with an average fragment area of 0·6 (sd 0·1) mm2 per g, while short chewing (11 s) generated 4342 (sd 1088) fragments per g with an average fragment area of 0·9 (sd 0·2) mm2 per g. Long chewing (24 s) of vegetarian chicken generated more than twice the number of bolus fragments (1079 (sd 278) per g for 24 s; 478 (sd 173) per g for 11 s) than short chewing (11 s). Increasing chewing time of vegetarian chicken from 11 to 24 s decreased bolus fragment area from 5·7 (sd 1·3) mm2 to 3·2 (sd 0·7) mm2 per g. Longer chewing of chicken led to a significantly larger increase in number of particles (P = 0·001) but a smaller decrease in mean area than vegetarian chicken. Bolus of chicken had significantly more (P < 0·001) and smaller (P < 0·001) particles than vegetarian chicken. Sex and nationality did not significantly (P > 0·05) affect number and area of bolus particles, suggesting that all participants followed the chewing instructions given by video well minimising inter-individual variation in oral behaviour.
Influence of chewing time and bolus properties on gastric and intestinal in vitro protein hydrolysis
Fig. 1 shows in vitro gastric protein DH% of chicken and vegetarian chicken chewed for 11 and 24 s.

Fig. 1.
In vitro gastric degree of protein hydrolysis (DH%) of chicken (a) and vegetarian chicken (b). (a) Reported as means and standard deviations of n 16 subjects. (b) Reported as means and standard deviations of n 15 subjects. (a) , Chicken chewed for 24 s;
, chicken chewed for 11 s. (b)
, Vegetarian chicken chewed for 24 s;
, vegetarian chicken chewed for 11 s.
In vitro gastric protein hydrolysis significantly increased with digestion time (P < 0·001). Chewing time had a significant impact on in vitro gastric protein digestion. The absolute difference between DH% after gastric digestion of proteins between long and short chewing times (DH%24s − DH%11s, t = 120 min) was significant and small, 2·1 % for chicken (Fig. 1(a)) and 1·0 % for vegetarian chicken (Fig. 1(b)). For both foods, long chewing time (24 s) resulted in significantly higher degree of protein hydrolysis than short chewing time (11 s) (P < 0·001), which is in line with our hypothesis (see the Introduction section). In addition, chicken has a significantly higher degree of protein hydrolysis than soya-based vegetarian chicken (P < 0·001). DH% value (t = 120 min) of chicken is 1·7 % higher (10·1 v. 8·4 %) for short chewing time (11 s) and 2·8 % higher (12·2 v. 9·4 %) for long chewing time (24 s) than vegetarian chicken.
Fig. 2 shows in vitro intestinal protein hydrolysis profiles (DH%) of chicken and vegetarian chicken chewed for 11 and 24 s. Table 3 shows the results of the statistical data analysis of in vitro gastric (n 16) and intestinal (n 7) protein DH% obtained by the linear mixed models.

Fig. 2.
In vitro intestinal degree of protein hydrolysis (DH%) of chicken (a) and soya-based vegetarian chicken (b). The DH% values for in vitro intestinal digestion at 0 min correspond to DH% values for in vitro gastric digestion at 120 min. Data are reported as means and standard deviations of n 7 subjects. (a) , Chicken chewed for 24 s;
, chicken chewed for 11 s. (b)
, Vegetarian chicken chewed for 24 s;
, vegetarian chicken chewed for 11 s.
Table 3. Effects of food matrix, chewing time, digestion time, sex and interaction of food matrix × chewing time on in vitro gastric (n 16) and intestinal (n 7) degree of protein hydrolysis (DH%) derived from linear mixed models

* P < 0·05, *** P < 0·001.
DH% significantly increased in absolute terms (Fig. 2 and Table 3, DH%120 min − DH%0 min, P < 0·001) from gastric to intestinal phase for chicken chewed for 11 s (76·5 − 11·8 % = 64·7 %), chicken chewed for 24 s (89·1 − 16·7 % = 72·4 %), vegetarian chicken chewed for 11 s (57·0 − 6·7 % = 50·3 %) and vegetarian chicken chewed for 24 s (69·6 − 10·7 % = 58·9 %). The differences of in vitro protein hydrolysis between chewing time of 11 and 24 s were enlarged after intestinal digestion for both foods. For chicken, the average protein hydrolysis difference between chewing time of 11 and 24 s (DH%24s − DH%11s, Fig. 2(a)) increased from 4·9 % (t = 0 min) to 12·6 % (t = 120 min), so almost by a factor of 2·5× during the intestinal phase (P = 0·013). Similarly, the average protein hydrolysis difference between chewing time of 11 and 24 s (Fig. 2(b)) increased from 4·0 % (t = 0 min) to 12·6 % (t = 120 min) for vegetarian chicken, so by a factor of 3·2× during the intestinal digestion (P = 0·049). Chewing time had a significant impact on in vitro intestinal protein digestion (Table 3). Long chewing (24 s) resulted in significantly higher intestinal degree of protein hydrolysis (89·1 % for chicken and 69·6 % for vegetarian chicken) than short chewing (11 s) (76·5 % for chicken and 57·0 % for vegetarian chicken). Chicken had a significantly higher degree of protein hydrolysis than vegetarian chicken (P < 0·001). DH% (t = 240 min) of chicken was 19·5 % higher for short chewing time (11 s) and 19·5 % higher for long chewing time (24 s) than vegetarian chicken. As evident in Table 3, sex, as a fixed effect (P = 0·64), and nationality, as a random factor, did not significantly affect in vitro gastric degree of protein hydrolysis, suggesting again that inter-individual variability in chewing behaviour was limited and subjects followed the chewing instructions provided by the video well. Therefore, during the study of in vitro intestinal protein digestion, the influence of sex and nationality were not considered. During both in vitro gastric and intestinal protein digestion, there was no interaction between food matrix and chewing time (P = 0·26; P = 0·74), indicating that the effect of chewing time was independent from the food matrix. In addition, no significant correlations (P > 0·05) were found between bolus properties (number, mean area) and in vitro protein gastric and intestinal hydrolysis of neither test foods (chicken, vegetarian chicken) under any chewing time (11 s, 24 s) with only one exception for the relation between mean area and gastric DH% of chicken (r −0·476, P = 0·006) (Table 4).
Table 4. Relationships between bolus properties (number of bolus particles, mean area of bolus particles) and in vitro protein gastric and intestinal digestibility (degree of hydrolysis; DH%) of chicken and vegetarian chicken derived by bivariate Pearson correlation (two-tailed)
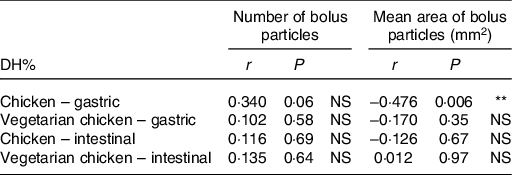
** P < 0·01.
Discussion
In the present study, we investigated the effect of naturally occurring oral processing behaviour on bolus properties and in vitro degree of protein hydrolysis of chicken and soya-based vegetarian chicken. The results demonstrated that there is a large inter-individual variation in natural chewing time for both foods and slow and fast eaters differed in chewing time by a factor of around 2×. Differences in natural oral processing behaviour lead to differences in bolus particle size and surface area as well as the differences in in vitro degree of protein hydrolysis of chicken and vegetarian chicken.
The first part of the present study determined natural chewing time of chicken and soya-based vegetarian chicken. Individual variations in oral processing behaviour such as chewing time have been reported previously while chewing frequency was similar between consumers(Reference Ranawana Viren, Henry and Pratt Megan6,Reference Woda, Mishellany and Peyron21) . The results of the cluster analysis showed that consumers with similar age, health condition and dental status differ by a factor of 2× in chewing time but did not differ considerably in chewing frequency, which is consistent with previous findings. These observations highlight the existence of large variations in chewing time within a fairly homogeneous population of similar age, health and dental status. Thus, in the present study, two substantially different chewing times for chicken and vegetarian chicken (11 and 24 s) were chosen as realistic measures of naturally occurring differences in chewing behaviour.
Bolus properties (number and mean area of bolus particles) of two test foods showed significant correlations with chewing time. For both foods, longer chewing time produced a larger number of smaller bolus particles (Table 2). It has been demonstrated that extended oral processing reduces bolus particle size of solid food(Reference Hutchings and Lillford22–Reference Boland24). One previous study reported that increasing chewing time of sausages resulted in an increase in number and decrease in size of sausage bolus fragments(Reference Devezeaux, Lavergne and Derks25). Therefore, our observation is in agreement with previous findings.
The second part of the present study was aimed at investigating the role of oral processing behaviour in in vitro protein digestion. Digestion is a complex series of mechanical and enzymatic processes wherein food matrix is physically and chemically disintegrated. Food particle size is mainly reduced during the oral and gastric digestion phase. During the intestinal digestion phase, enzymatic hydrolysis and nutrient absorption occur(Reference Guerra, Etienne-Mesmin and Livrelli26,Reference Do, Singh and Oey27) . In both gastric and intestinal digestion, long chewing resulted in a higher in vitro protein DH% than short chewing for both test foods (Figs. 1 and 2). As a consequence of longer chewing, the food matrix is disintegrated into more and smaller bolus fragments (Table 2) thereby generating a larger overall surface area, which increases the accessibility of gastric fluids carrying H+ and pepsin to the inner core of the bolus particles(Reference Golding, Gouseti, Bornhorst, Bakalis and Mackie28). This finding is consistent with our initial hypothesis (see the Introduction section) and supported by previous studies(Reference Suzuki, Fukushima and Okamoto5,Reference Ranawana Viren, Henry and Pratt Megan6,Reference Cassady, Hollis and Fulford12,Reference Pennings, Groen and Van Dijk15,Reference Kong and Singh29) . Nevertheless, some limitations of the present methodological approach must be mentioned. Although the oral phase was conducted as in vivo human mastication which ensured the authenticity of bolus at the beginning of in vitro gastric digestion, bolus properties were determined on an aliquot representing 20 % w/w of each bolus (2 × 0·5 g of around 5 g bolus). This may have had an impact on the representativeness of the boli samples. In addition, in vitro gastric digestion was conducted under constant-rate rotation mixing conditions, whereas the actual driving force of food bolus disintegration in the stomach is peristaltic movement of stomach walls(Reference Kong and Singh29,Reference Kong and Singh30) . Therefore, the disintegration behaviour of a compact and cohesive bolus, and consequently the acid hydrolysis and enzymatic hydrolysis of nutrients during digestion may have been different in our simulated in vitro experiment compared with the in vivo situation. The difference in in vitro degree of protein hydrolysis induced by chewing time was enlarged after intestinal digestion for both foods. For the most extreme cases, the differences increased by a factor of 7·9× for chicken and 20·2× for vegetarian chicken. The potential physiological relevance of these differences is worth to be discussed. The INFOGEST method used herein is a static model which is inadequate for simulation of some dynamic aspects of digestion including pH gradients, gradual secretion of gastric fluid and enzymes as well as gastric emptying(Reference Brodkorb, Egger and Alminger16). Hence, these findings should be considered with caution. However, if the influence of oral processing caused such a large difference in in vitro protein digestion, it gives a hint for the effective involvement of oral processing behaviour in in vivo protein digestion and even further utilisation.
Although longer chewing generated more and smaller bolus particles and higher in vitro degree of protein hydrolysis for both test foods, no correlation was observed between bolus properties (number and mean area of bolus particles) and in vitro gastric or intestinal protein hydrolysis in the current study. Although there was one exception for the relation between mean area of bolus particle and gastric DH% of chicken, it was still insufficient to generalise a correlation between bolus properties and in vitro degree of protein hydrolysis. Previous studies reported inverse correlations between particle size and in vivo starch hydrolysis rate of rice but not spaghetti(Reference Ranawana Viren, Henry and Pratt Megan6), which suggests that the intrinsic status (e.g. cell wall) and processing status (e.g. milling) of the food may affect subsequent starch digestion. Considering the consumption of plant foods, cereals (e.g. rice) and legumes (e.g. common beans) exhibit a cellular microstructure in which cell walls play a role as barriers for digestive enzyme diffusion to bolus particles(Reference Ranawana Viren, Henry and Pratt Megan6,Reference Zahir, Fogliano and Capuano19,Reference Pallares, Loosveldt and Karimi31) . Therefore, the inverse correlation between particle size and starch digestibility hinges on the fact that the smaller the particles, the more intact cells of food matrix are disintegrated and thereby the more the exposure of nutrients to enzymes as well as the shorter path of enzymes to the core of particles. The lack of correlation between bolus properties (number and mean area of bolus particles) and in vitro gastric or intestinal protein hydrolysis in our study may have been caused by less severe resistance to enzyme diffusion in chicken or soya-based vegetarian chicken since there is no barrier effect of cell walls. On top of that, in the study on rice and spaghetti, the correlations between particle size and starch digestion (incremental AUC) were only carried out with a certain range of particle sizes (>2000 μm and <500 μm) and were significant only until 60 min of intestinal digestion(Reference Ranawana Viren, Henry and Pratt Megan6), whereas in our study, correlations were computed using number and mean area of bolus particles and DH% only at the end of gastric and intestinal digestion. Moreover, if the hypothesis is correct that the rate of digestion depends on the accessibility of digestive enzymes to the substrates within particles, then nutrient digestion would be proportional to the particle surface area relative to its volume across the whole range of bolus particles. In other words, the accessibility of enzymes to their substrate may have not been fully described by just the mean particle area and a more sophisticated indicator which would consider the surface to volume ratio for each particle must be used. In addition, our data used for correlation analysis are not very powerful since only four bolus samples (chicken and vegetarian chicken at 11 and 24 s) were collected to test for correlations between bolus properties and protein digestibility. Admittedly, these data points are not continuously covering the whole range of bolus particle number and bolus mean area values but are clustered around the mean values of each treatment, which makes the correlation less powerful. Therefore, the number and mean area of bolus particles as computed in the present study could be good indicators of the degree of oral processing but not the most reliable factors for predicting bolus gastrointestinal digestibility. It is also possible that other bolus properties such as level of cohesion of the bolus particles may play a role in protein digestion by modulating the accessibility of enzymes to proteins.
When comparing the chicken with the soya protein-based vegetarian chicken, we observed that chicken always generated more and smaller bolus fragments and higher in vitro degree of protein hydrolysis than soya-based vegetarian chicken regardless of chewing time. Oral processing behaviour is influenced by food texture and subsequently influences bolus properties(Reference Mioche, Bourdiol and Peyron1,Reference Chen, Chen and Engelen23,Reference Chen32) . Therefore, the differences in bolus properties between chicken and soya-based vegetarian chicken might be related to differences in the texture of the foods. Most plant-based proteins have lower protein digestibility than animal proteins. However, previous studies reported that the highest in vitro degree of protein hydrolysis for chicken was 92·0 %(Reference Carbonaro, Maselli and Nucara33). For soya protein concentrate, degree of protein hydrolysis in vivo was 95·0–97·0 % which was comparable with the digestion of animal proteins(Reference Hughes, Ryan and Mukherjea34,35) . In the current study, the highest in vitro degree of protein hydrolysis for chicken was 89·1 % (24 s) which was similar to the previous study, while for vegetarian chicken this was 69·6 % (24 s), i.e. relatively lower compared with isolated proteins. This might be a complex and multi-factorial consequence of the effect of the food matrix on protein digestion. Chicken breast meat is mainly composed of myosin which is the most pre-dominant myofibrillar component(Reference Liu and Xiong36). Vegetarian chicken used in the present study is composed of soya protein concentrate (93 %). Two major protein fractions of soya protein concentrate are storage globulins 7S (β-conglycinin) and 11S (glycinin)(Reference Joshi and Kumar37–Reference Sarkar, Singh, Chen and Engelen39). The difference of major storage proteins between chicken and vegetarian chicken could be a factor that can affect the difference in in vitro protein digestion(Reference Carbonaro, Maselli and Nucara33). From a microstructural point of view, it is obvious that chicken breast meat is organised in a fibrous structure. We could observe visually a meat-like structure with presence of fibres also in the vegetarian chicken although we did not investigate how the vegetarian chicken proteins were organised at the microscopic level. Fibrous meat analogues are typically obtained through extrusion of protein blends that generates fibrous structures by cross-linking of proteins(Reference Dekkers, Boom and van der Goot40). The fibre formation process might have increased cross-links and leads to aggregation of proteins in the vegetarian chicken, which can reduce protein susceptibility to enzymatic proteolysis(Reference Kaur, Maudens and Haisman41). Moreover, mild cooking of chicken is known to improve digestion of myosin heavy chain by denaturation(Reference Liu and Xiong36,Reference Kaur, Maudens and Haisman41) . This may explain the relatively high in vitro degree of protein hydrolysis of chicken. Additionally, it is well known that the presence of anti-nutrients may reduce digestibility of plant-based proteins including soybean proteins(Reference Joshi and Kumar37,Reference Arntfield and Yada38) . However, the vegetarian chicken was probably highly processed during production and cooked before the chewing sessions. It is reasonable to believe that such anti-nutritional factors should have been removed and inactivated during processes such as dehulling, protein extraction, isolation and heating(Reference Arntfield and Yada38). Interestingly, fibres are present in the vegetarian chicken as reported in the nutrition facts. Fibre supplementation has been reported to decrease protein digestibility(Reference Capuano42). However, we have no information about the type of fibre present in the soya-based vegetarian chicken, so it is difficult to speculate about the contribution the fibres may have on in vitro protein hydrolysis. Another explanation for the difference in in vitro degree of protein hydrolysis might be the difference in bolus properties after oral processing. As mentioned above, chicken is further broken down into more and smaller bolus fragments than vegetarian chicken (Table 2) thereby providing larger overall surface area after the same chewing time. This might increase the susceptibility of acidic hydrolysis and enzymatic proteolysis of food matrix(Reference Golding, Gouseti, Bornhorst, Bakalis and Mackie28).
A strength of the current study is the efficient design of the combination of in vivo oral processing and in vitro gastrointestinal digestion. The results facilitate understanding of the relations between natural oral processing and protein digestion. One limitation of the study was that it was difficult to choose a practical method to accurately determine the whole range of sizes of bolus particles. This might influence the assessment of correlations between particle size distribution and in vitro protein digestion. Another limitation is that in vitro data did not include gastric peristalsis and emptying(Reference Brodkorb, Egger and Alminger16,Reference Kong and Singh30) . However, this in vitro study may set the stage for future in vivo studies to validate whether the observed effects are physiologically relevant.
In conclusion, the present study demonstrated that natural chewing time of chicken and soya-based vegetarian chicken varies considerably between consumers. Longer chewing leads to more and smaller particles in food bolus and increases in vitro degree of protein hydrolysis. Regardless of the effect of chewing time, in vitro degree of protein hydrolysis of chicken was always higher than soya-based vegetarian chicken. Oral processing is the very first stage of food digestion of which the behaviour can be manipulated consciously by humans. The present study heightens the understanding of how differences in natural oral processing behaviour translate into differences in nutrient digestion. Further research is needed to fully understand the relationships between oral processing behaviour and nutrient digestion as well as which boli properties are predictive of protein digestion in the gastrointestinal tract. Further studies are needed to reveal whether inter-individual differences in food oral processing behaviour contribute to inter-individual differences in macronutrient digestion. In addition, the degree of disintegration of undigested proportion of food fragments in the stomach and small intestine may influence the nutrient fermentation in the gut, which might be another interesting direction of research.
Acknowledgements
The authors would like to thank Junhao Li, Sijie Mei and Jelmer Hamer for their help with the execution of the experimental study and data analysis.
The author Y. C. received a PhD scholarship from the China Scholarship Council (CSC no. 201807720020).
The authors’ responsibilities were as follows: Y. C., M. S. and E.C. designed the research; Y. C. conducted the research; Y. C. analysed data; Y. C., M. S. and E. C. wrote the paper; Y. C. had primary responsibility for final content; all authors read and approved the final manuscript.
There are no conflicts of interest to declare.