The human intestine contains a dense and complex community of microbes, and many representatives permanently inhabit this niche( Reference Claes, Garcia and Lebeer 1 , Reference van Baarlen, Kleerebezem and Wells 2 ). In addition to the resident microbiota, various bacteria transiently pass through the intestine as a consequence of ingestion – for example in fermented food products, or as supplements containing probiotics( Reference Bron, van Baarlen and Kleerebezem 3 , Reference Cani 4 ). The latter are defined as ‘live micro-organisms that, when administered in adequate amounts, confer a health benefit on the host’( Reference Hill, Guarner and Reid 5 , 6 ). In the intestinal tract, a single layer of epithelial cells forms a physical barrier between the intestinal lumen, the lamina propria and the mucosal-associated lymphoid tissue. In addition, mucus secreted by goblet cells in the epithelium serves to spatially compartmentalise the bacteria to the lumen and prevent bacterial colonisation of the epithelium( Reference Johansson, Larsson and Hansson 7 – Reference Vaishnava, Yamamoto and Severson 9 ). Mice lacking Muc2, the major secreted mucin in the intestine, develop spontaneous colitis demonstrating its important barrier function( Reference Van der Sluis, De Koning and de Bruijn 10 ). Mucosal Ig and antimicrobial peptides (AMP) and proteins secreted by the epithelial cells serve to enhance the barrier function of the epithelium through immune exclusion and killing of bacteria( Reference Rossi, van Baarlen and Wells 11 ). Moreover, in mucus-deficient mice, the ileal region of the intestine appears to be protected from excessive inflammatory responses by activation of epithelial repair and barrier maintenance pathways through the IL-22-STAT regulatory axis( Reference Sovran, Loonen and Lu 12 ).
The first direct evidence for the role of epithelial integrity in preventing intestinal inflammation came from a chimeric mouse model in which parts of the small bowel epithelium expressed N-cadherin instead of E-cadherin, thereby disrupting the E-cadherin homotypic interactions that help maintain junctional integrity( Reference Hermiston and Gordon 13 ). The regions of the intestine expressing N-cadherin were more permeable than the areas expressing E-cadherin and developed focal lesions because of inflammation. Since then, studies in several different knockout mice have shown that defects in the pathways maintaining epithelial integrity, epithelial stress responses or the regulation of mucosal immune responses lead to barrier destruction and colitis triggered by bacterial antigens and microbe-associated molecular patterns (MAMP)( Reference Takahashi, Vereecke and Bertrand 14 – Reference Welz, Wullaert and Vlantis 19 ).
Despite the role of commensal MAMP in triggering inflammatory responses, homeostasis is not maintained by a ‘passive’ mechanism of lack of recognition. In contrast, Toll-like receptor (TLR) activation by commensal bacteria was shown to play a crucial role in the recovery from epithelial damage induced by dextran sodium sulphate (DSS)( Reference Rakoff-Nahoum, Hao and Medzhitov 20 – Reference Rossi, Karczewski and Stolte 24 ). Similarly, mice with a knockout of NEMO (ikappa kinase γ), an activator of NF-κB, develop spontaneous colitis because of the role of NF-κB in inducing epithelial repair and innate effector mechanisms in the intestine( Reference Nenci, Becker and Wullaert 18 ). TLR signalling and in particular TLR-2 signalling and protein kinase C (PKC)α and PKCδ activation have also been implicated in tight-junction (TJ) function modulation and epithelial permeability( Reference Karczewski, Troost and Konings 25 ).
A limited number of studies report the impact of probiotics on barrier integrity in (healthy) human volunteers, although several plausible direct and indirect mechanisms of bacterial modulation of barrier function have been postulated (Fig. 1). For example, Gotteland et al. ( Reference Gotteland, Cruchet and Verbeke 26 ) evaluated the impact of consumption of Lactobacillus rhamnosus GG in healthy human volunteers upon epithelial barrier challenge by treatment with the nonsteroidal anti-inflammatory drug indomethacin. The authors reported that the consumption of live L. rhamnosus GG protected the integrity of the gastric mucosal barrier, but had no effect at the intestinal level( Reference Karczewski, Troost and Konings 26 ), highlighting the differences in physiology of the epithelial barrier in different locations of the gastrointestinal tract. Karczewski et al. ( Reference Karczewski, Troost and Konings 25 ) performed TJ staining in duodenal samples obtained from healthy volunteers after perfusion with Lactobacillus plantarum WCFS1, revealing that the TJ localisations of scaffold protein ZO-1 (zonula occludens-1, also known as tight junction protein-1) and the transmembrane protein occludin were significantly increased by the interaction with this bacterium. Subsequently, these authors showed that the same strain conferred protection against chemically induced barrier disruption in an in vitro Caco-2 cell line model, involving a TLR-2-dependent signalling pathways( Reference Karczewski, Troost and Konings 25 ). These studies imply that probiotic interventions are a plausible approach to enhance epithelial barrier function in human subjects, possibly in a location-specific (gastric v. intestinal) manner.
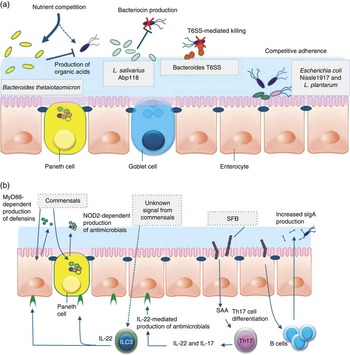
Fig. 1 Direct and indirect mechanisms by which commensals or probiotics can antagonise pathogens. Graphical display of exemplary direct (a) and indirect (b) mechanisms of barrier function modulation by bacterial (probiotic) interaction. (a) Direct mechanisms of barrier function modulation by interaction of commensals and probiotics. Bacteria can compete with enteric pathogens by competition for carbohydrates depending on the diet( Reference McNaught, Woodcock and Anderson 227 ). A probiotic strain of Lactobacillus salivarius UCC118 produces a bacteriocin in vivo that can significantly protect mice against infection with the invasive foodborne pathogen Listeria monocytogenes ( Reference Corr, Li and Riedel 36 ). In diverse Bacteroides species, a type VI secretion system (T6SS) mediates cell-contact-dependent mechanisms of inter-bacterial antagonism via the export antibacterial proteins( Reference Klaenhammer, Kleerebezem and Kopp 228 ). Probiotics have been shown to inhibit the colonisation of pathogens through competition for common receptors of adhesion( Reference Adlerberth, Ahrne and Johansson 48 ). (b) Indirect mechanisms of pathogen antagonism. Recognition of microbe-associated molecular patterns by host pattern recognition receptors such as TLR and NLR activate immune defences and protect against infection. TLR signalling induces expression of defensins in enterocytes and antimicrobial factors in Paneth cells via the myeloid differentiation primary response 88 (MYD88) activation of NF-κB leading to the production of antimicrobial peptides. Similarly, nucleotide-binding oligomerisation domain 2 (NOD2) recognition of bacterial peptidoglycan induces cryptdin expression by Paneth cells. Sensing of an unknown signal from commensal microbes stimulates type 3 innate lymphoid cells (ILC) to secrete IL-22, which signals through the receptor (IL-22R) on intestinal epithelial cells to increase expression of the fucosyltransferase (FUT), mucin expression and antimicrobials, including regenerating family member 3 (Reg3) proteins. Segmented filamentous bacteria (SFB) closely associate with the ileum epithelium and stimulate maturation of the B- and T-cell compartments leading to enhanced IgA production by B cells and serum amyloid A (SAA)-dependent T helper 17 (Th17) cell differentiation. Th17 cells produce inflammatory cytokines and IL-22, which stimulate innate immune defence mechanisms to fight off infections.
Increased mucosal permeability and loss of epithelial integrity are recognised to play a role in the pathophysiology of a variety of (gastrointestinal) disorders, including the challenge by intestinal pathogens, inflammatory bowel disease (IBD), irritable bowel syndrome (IBS), obesity and the metabolic syndrome and necrotising enterocolitis (NEC). One hallmark of all these disorders is the involvement of a compromised barrier function in the pathophysiology. Thus, many probiotic studies have been targeting patient populations or animal models for human diseases, to evaluate their protective capacity on clinical parameters, including barrier function. Below, we review the state of the art of these approaches and propose future strategies aiming to further substantiate our understanding of the mode of action of probiotics.
Pathogen challenges to the barrier
The mucosal barrier can be challenged by encounters with bacterial or viral pathogens; however, for obvious ethical reasons, it is not trivial to perform well-controlled human infection studies. As an alternative, attenuated pathogenic bacteria, which were developed as oral vaccines, have been used for experimental challenge studies in humans( Reference Davitt and Lavelle 27 ). The attenuated bacterial pathogens generally lead to mild diarrhoea, offering a model system for controlled enteric pathogen challenge studies in human individuals( Reference Harro, Chakraborty and Feller 28 , Reference Bovee-Oudenhoven, Lettink-Wissink and Van Doesburg 29 ). However, it remains unclear whether these (more or less) attenuated enteric pathogens have similar effects on the mucosal barrier function as compared with the pathogenic strains they were derived from. Nevertheless, these models have been used to study the protective capacity of probiotics and other dietary ingredient interventions, with generally poor success rates for probiotics in the amelioration of diarrhoea symptoms( Reference Ten Bruggencate, Girard and Floris-Vollenbroek 30 , Reference Ouwehand, Ten Bruggencate and Schonewille 31 ). Several studies have evaluated the potential of probiotics in eradication therapy of Helicobacter pylori ( Reference Li, Huang and Sui 32 ) and the prevention of rotavirus-caused diarrhoea in children( Reference Saavedra, Bauman and Perman 33 , Reference Szajewska, Wanke and Patro 34 ), although with a variable outcome.
Rodent models offer more flexibility and control for challenge studies with enteric pathogens and in examining the potential of probiotics to affect the efficacy of infection. In this area, various rodent in vivo ( Reference Bujalance, Jimenez-Valera and Moreno 35 ) infection models have demonstrated that probiotics can attenuate the severity of infection caused by several gastrointestinal pathogens, including H. pylori, Citrobacter rodentium, Listeria monocytogenes and Salmonella typhimurium ( Reference Corr, Li and Riedel 36 – Reference Zoumpopoulou, Foligne and Christodoulou 41 ). In many cases, the molecular mechanisms behind the positive effects remain unknown. Various mechanisms of probiotic action have been postulated that can explain their positive effects in enteropathogen infection models, although in only few of these scenarios there is a direct and pivotal role established for their effect on mucosal barrier function( Reference Bujalance, Jimenez-Valera and Moreno 35 ).
The presence of commensal bacteria can inhibit the efficacy of enteropathogens via ‘colonisation resistance’, which encompasses a variety of molecular mechanisms involving microbe–microbe and/or microbe–host interactions( Reference Spees, Lopez and Kingsbury 42 , Reference Derrien and van Hylckama Vlieg 43 ). Ingested probiotic bacteria may modulate the endogenous microbiota composition by competing for (micro-)nutrients and/or the production of (secondary) metabolic products, thereby modulating the endogenous microbiota composition and/or activity, which may affect colonisation resistance and risk or severity of enteric pathogen infection( Reference Derrien and van Hylckama Vlieg 43 ).
Probiotics can produce antimicrobial compounds that may have an impact on the pathogenic bacteria directly, or via their effects on the endogenous microbiota. For example, Lactobacillus reuteri can produce reuterin, a secondary metabolite with broad-spectrum antimicrobial activity that can modulate the microbiota composition, as well as directly inhibit enteric pathogens( Reference Cleusix, Lacroix and Vollenweider 44 ). Alternatively, probiotics may produce broad- or narrow-spectrum bacteriocins, which are small ribosomally synthesised peptides that inhibit the growth of other bacteria( Reference Cotter, Hill and Ross 45 ), which may affect enteric pathogens directly. A well-developed example of such bacteriocin is the class II bacteriocin Abp118 that is produced by Lactobacillus salivarius UCC118 and effectively inhibits L. monocytogenes infection and barrier disruption in vivo in a mouse model( Reference Corr, Li and Riedel 36 ). Probiotics may also directly compete for mucosal adherence sites with pathogenic bacteria, a mechanism termed competitive exclusion( Reference Servin 46 ). However, in many cases the mechanism of anti-adherence remains unclear. A mannose-specific adhesin of L. plantarum has been identified that is proposed to play a role in the competitive exclusion of FimH-dependent adhesion of enteropathogenic Escherichia coli (EPEC), or other mannose adhesion-dependent enteric pathogens (e.g. S. typhimurium)( Reference Pretzer, Snel and Molenaar 47 , Reference Adlerberth, Ahrne and Johansson 48 ). Besides such direct competitive epithelial binding, other mechanisms may also underlie competitive exclusion such as the degradation of mucosal surface receptors by secreted enzymes, or the production of receptor analogues or bio-surfactants. However, in light of recent insights about the mucus barrier, the in vivo relevance of in vitro studies evaluating the inhibition of pathogen adherence to cell lines might be questioned. This is because of the fact that commensal contact with epithelial cells is very limited and hardly observed in vivo, because of the presence of a physical mucus barrier. In the colon, a thick firm layer of mucus overlays the epithelium and is devoid of bacteria, despite the large numbers residing in the lumen( Reference Jakobsson, Rodriguez-Pineiro and Schutte 49 ). In the small intestine, the mucus layer is thinner, but bacteria are rarely seen in contact with the epithelium( Reference Loonen, Stolte and Jaklofsky 8 ), the exception being segmented filamentous bacteria in mice that have an atypical and intimate association with enterocytes( Reference Klaasen, Koopman and Poelma 50 ). In contrast to gram-positive probiotic strains, several enteric gram-negative pathogens possess flagella, which propel them through mucus. The probiotic E. coli Nissle (EcN) is flagellated and motile and thus has increased potential to inhibit pathogen adherence and invasion. EcN has been shown to inhibit invasion of various epithelial cell lines by Yersinia enterocolitica, Shigella flexneri, S. typhimurium and L. monocytogenes, via secretion of an unidentified factor rather than competition for receptors( Reference Altenhoefer, Oswald and Sonnenborn 51 ).
Many pathogens influence barrier permeability through production of toxins that disrupt TJ and/or cause increased secretion or decreased absorption of fluids and electrolytes( Reference Lu, Yang and Hu 52 , Reference Hodges and Gill 53 ). Bifidobacterium breve strain Yakult and Bifidobacterium pseudocatenulatum DSM20439 have been described to inhibit the expression of Shiga toxin in enterohemorrhagic E. coli (EHEC) 0157:H7 strains in vitro, as well as in vivo in a mouse infection model( Reference Asahara, Shimizu and Nomoto 54 ). This effect appears relatively unspecific, as subsequent work illustrated Shiga toxin expression inhibition in EHEC in vitro by fifteen different probiotics, depending on their capacity to produce organic acids( Reference Carey, Kostrzynska and Ojha 55 ). A more specific mechanism has been proposed for probiotic yeast Saccharomyces boulardii that secretes a protease that can degrade Toxin A that is produced by Clostridium difficile ( Reference Castagliuolo, LaMont and Nikulasson 56 ). Lesions in the epithelium can also be caused by cytoskeleton rearrangements induced by colonisation and invasion of enteropathogens. In vitro, these virulence mechanisms cause a drop in transepithelial electrical resistance (TEER) of epithelial cell monolayers. Lactobacillus strains of intestinal origin have been shown to inhibit pathogen-induced disruption of epithelial integrity. Lactobacillus acidophilus LB( Reference Lievin-Le Moal, Amsellem and Servin 57 ) and Lactobacillus helveticus R0052( Reference Sherman, Johnson-Henry and Yeung 58 ) have been shown to antagonise the cytoskeleton rearrangements caused by EPEC in vitro. Similar studies have shown that L. helveticus strain R0052( Reference Sherman, Johnson-Henry and Yeung 58 ) L. plantarum 299v( Reference Mangell, Nejdfors and Wang 59 , Reference Michail and Abernathy 60 ) and Lactobacillus casei DN-114 001( Reference Parassol, Freitas and Thoreux 61 ) reduce the drop in TEER caused by infection of T84 intestinal cells with EHEC and EPEC.
More recently, the concept of indirect pathogen antagonism arose by the recognition that microbial colonisation can stimulate innate and adaptive immune responses in the intestinal mucosa( Reference Rossi, van Baarlen and Wells 11 , Reference Wells, Rossi and Meijerink 62 ). Stimulation of innate receptors expressed in the epithelium can enhance the production of inducible antimicrobials such as epithelial β-defensins and members of the antimicrobial C-type lectins in the gut( Reference Everard, Geurts and Caesar 63 ). Indeed, colonisation of germ-free mice and the expansion of bacteria after weaning caused an increased regenerating family member 3 (Reg3) production( Reference Cash, Whitham and Behrendt 64 , Reference El Aidy, van Baarlen and Derrien 65 ). However, some studies reported no change in production of defensins by commensal colonisation of the gut( Reference Putsep, Axelsson and Boman 66 , Reference Hase, Eckmann and Leopard 67 ), which may be due to differences in the regulatory control of certain AMP. Several studies using epithelial cell lines in vitro have demonstrated the capacity of probiotics to differentially regulate defensins( Reference Paolillo, Carratelli and Sorrentino 68 – Reference Wehkamp, Harder and Wehkamp 71 ). Apart from the induction of AMP, probiotics might affect the mucus barrier. This could be through bacterial interaction with innate cells in the mucosa producing cytokines (e.g. IL-22( Reference Rossi, van Baarlen and Wells 11 ), IL-8( Reference McGuckin, Linden and Sutton 72 )) that can increase the expression of specific mucin genes. For example, in vitro studies have shown that adherent Lactobacillus spp. can stimulate MUC3 expression in human intestinal epithelial cells and thereby inhibit EPEC adherence( Reference McGuckin, Linden and Sutton 72 – Reference Mack, Michail and Wei 74 ). Analogously, lipopolysaccharide (LPS) can stimulate expression and secretion of MUC5AC and MUC5B( Reference Smirnova, Guo and Birchall 75 ), and thereby enhance mucus barrier function( Reference Smirnova, Guo and Birchall 75 ). These examples illustrate the potential of probiotics to enhance epithelial and mucus barrier functions, thereby protecting the host against enteric pathogens.
Obesity and diabetes
Low-grade inflammation, and in particular endotoxaemia (i.e. relatively high amounts of gut microbiota-derived LPS in plasma), has been identified as a factor involved in the onset and progression of metabolic diseases associated with obesity (insulin resistance, type 2 diabetes) in human and rodent models( Reference Cani, Possemiers and Van de Wiele 76 – Reference Wellen and Hotamisligil 79 ). A high fat content in the diet and changes in barrier function are considered to be important factors in endotoxaemia( Reference Laugerette, Vors and Geloen 80 , Reference Erridge, Attina and Spickett 81 ). Several studies have shown that both diet-induced and genetic obese and type 2 diabetic mice displayed changes in gut microbiota( Reference Cani, Possemiers and Van de Wiele 76 , Reference Cani, Amar and Iglesias 77 , Reference Cani, Bibiloni and Knauf 82 – Reference Waldram, Holmes and Wang 86 ). Among the reduced abundance bacterial groups were the bifidobacteria( Reference Cani and Delzenne 87 ), which in mice has been associated with reduced intestinal LPS levels and improved mucosal barrier function( Reference Wang, Yao and Xiao 88 – Reference Wang, Yu and Ochani 92 ). These observations are in agreement with the reduction of metabolic endotoxaemia, gut barrier dysfunction and inflammatory disorders that could be achieved in mice by prebiotic supplementation, which restored the bifidobacterial abundance in the intestinal microbiota( Reference Cani, Possemiers and Van de Wiele 76 , Reference Cani, Neyrinck and Fava 83 , Reference Muccioli, Naslain and Backhed 93 , Reference Pachikian, Neyrinck and Deldicque 94 ). However, the precise mechanism underlying these health improvements remain unclear, and may not be entirely attributed to Bifidobacterium spp.( Reference Everard, Lazarevic and Derrien 95 ). Although rodent models have revealed the impact of prebiotic supplementation on obesity, type 2 diabetes and gut barrier function( Reference Delzenne and Cani 96 – Reference Cani and Delzenne 99 ), only few studies have been conducted in human obese and type 2 diabetic populations( Reference Parnell and Reimer 100 – Reference Dewulf, Cani and Claus 102 ). One of these studies described enhanced weight loss and improved glucose regulation in obese adults who consumed fructo-oligosaccharide (FOS) supplements (21 g/d) for 12 weeks, which was tentatively associated with FOS-induced suppressed ghrelin and enhanced peptide YY production( Reference Parnell and Reimer 100 ). The second study showed that long-term synbiotic supplementation (Bifidobacterium longum and FOS) in sixty-six overweight non-alcoholic steatohepatitis patients could reduce endotoxaemia, insulin resistance, steatosis and several metabolic and inflammatory parameters( Reference Malaguarnera, Vacante and Antic 101 ). Besides these studies using prebiotic and synbiotic supplementation in rodent models and human populations, several studies have reported on the beneficial impacts of probiotics on obesity and type 2 diabetes in rodent models. In many of these studies, diet-induced obesity models were used to induce metabolic dysfunction in the animal models to subsequently address the possible health beneficial role of probiotic supplementation( Reference Cani and Van Hul 103 ). In such a setting, various lactobacilli were shown to positively affect various metabolic disorder parameters. For example, Lactobacillus gasseri BNR17 reduced body weight and fat mass development in overweight rats( Reference Yun, Park and Kang 104 , Reference Kang, Yun and Park 105 ), whereas in high-fat-diet-induced obese mouse models L. plantarum 14 supplementation was shown to reduce the mean adipocyte size( Reference Takemura, Okubo and Sonoyama 106 ) and Lactobacillus paracasei F19 could induce a reduction of total fat mass and plasma TAG possibly via a mechanism involving angiopoietin-like factor 4( Reference Aronsson, Huang and Parini 107 ). In contrast, L. acidophilus NCDC supplementation in diet-induced obese mice did not induce any significant changes in body fat composition and/or liver and muscle fat content( Reference Arora, Anastasovska and Gibson 108 ). Analogously, L. casei Shirota did not affect fat mass and body weight in diet-induced obese mice but, nevertheless, did induce a reduction of insulin resistance and endotoxaemia, illustrating that the impact on gut barrier function and glucose regulation can be elicited by mechanisms that are independent of body weight and fat mass development( Reference Naito, Yoshida and Makino 109 ). A few human intervention studies were also performed, including a study that suggests that specific Lactobacillus strains have an impact on body fat, weight and metabolic disorders. For example, the ingestion of L. gasseri SBT2055 for 12 weeks reduced fat-mass gain, body weight, waist:hip ratio in overweight subjects compared with placebo( Reference Kadooka, Sato and Imaizumi 110 ), an effect recently confirmed by the authors( Reference Kadooka, Sato and Ogawa 111 ). Jung et al.( Reference Jung, Lee and Kang 112 ) have shown that the ingestion of Lactobacillus gasseri BNR17, a strain showing beneficial effects in rodents, reduced weight and waist and hip circumference after 12 weeks of treatment. Sanchez et al. have observed that supplementation with L. rhamnosus CGMCC1.3724 (1·6×108 colony-forming units (CFU) of the strain per capsule with oligofructose and inulin) helped to lose more weight in women after the first 12 weeks, whereas it has no effect in men. Interestingly, while women in the placebo group regain weight during the weight-maintenance period, opposite changes were observed in women from the treated group who continued to lose body weight and fat mass. Finally, another study showed that the perinatal modulation of the gut microbiota by L. rhamnosus GG reduces excessive weight gain during the first years of life in children( Reference Luoto, Kalliomaki and Laitinen 113 ), thereby suggesting that perinatal intervention may also be beneficial for the infants.
Several studies described a positive impact of Lactobacillus spp. supplementation on diet-induced metabolic disorders, including improved intestinal barrier function and reduced endotoxaemia. However, a recent study showed that in 3-week-old mice the high-dosage supplementation with Lactobacillus ingluviei (4×1010 CFU) led to increased overall weight gain, as well as total liver weight, while not affecting fat mass despite increased transcription levels of the lipogenic markers (PPARγ and sterol regulatory element-binding protein 1c) in the gonadal fat mass( Reference Angelakis, Bastelica and Ben Amara 114 ). The variability in the results obtained in these models imply that both the specific rodent model (age of the animals and the mode by which metabolic disorders are induced) and the choice of Lactobacillus spp. could have a major impact on the outcomes of these interventions. Nevertheless, in adult animals there are multiple indications that lactobacilli may positively affect different metabolic disorder parameters, including compromised barrier function and inflammatory status.
Analogous to the lactobacilli, bifidobacteria have also been evaluated for their capacity to improve metabolic disorders in diet-induced rodent models for obesity and diabetes( Reference Cani and Van Hul 103 ). For example, in a high-fat-diet-induced obesity model in rats, a mixture of three Bifidobacterium strains (B. pseudocatenulatum SPM 1204, B. longum SPM 1205 and B. longum SPM 1207) was shown to reduce body weight and adiposity, but it also reduced cholesterol (total, HDL-cholesterol and LDL-cholesterol), TAG, glucose, leptin and hepatic enzyme (aspartate aminotransferase, alanine aminotransferase and lipase) levels in plasma, suggesting beneficial effects of this strain mixture in high-fat-diet-induced obesity and type 2 diabetes( Reference An, Park and Lee do 115 ). In a similar model, B. longum supplementation could compensate the decreased endogenous Bifidobacterium abundance induced by the high-fat diet, and led to reduced body weight gain, fat mass, insulin resistance, systolic blood pressure, as well as lowered metabolic endotoxaemia, and IL-1β and intestinal myeloperoxidase levels in plasma. The authors proposed that these effects might be mechanistically linked to enhanced barrier function induced by B. longum supplementation via increased expression of intestinal REG-family proteins( Reference Chen, Wang and Li 116 ). Notably, a recent study illustrated that in high-fat-diet-induced obese mice, the translocation of gram-negative Enterobacteriaceae to adipose tissue and blood was increased, which was accompanied by elevated inflammation status. This ‘metabolic bacteremia’ could be suppressed by dietary supplementation with Bifidobacterium animalis subsp. lactis 420, which not only normalised inflammation status but also corrected insulin sensitivity and hyperinsulinemia( Reference Amar, Chabo and Waget 117 ). Intriguingly, this Bifidobacterium strain was also shown to competitively exclude the binding of pathogenic bacteria to the mucosa( Reference Collado, Meriluoto and Salminen 118 ) and improve TJ integrity( Reference Putaala, Salusjarvi and Nordstrom 119 ) in vitro, again pointing towards enhanced intestinal barrier function as the compensatory mode of action.
More recently, the role of another putative beneficial bacterium, namely Akkermansia muciniphila, has been discovered. Obese and diabetic mice treated with A. muciniphila exhibit a reduced body weight and fat mass gain, a reduced inflammation and metabolic endotoxaemia upon high-fat-diet feeding. Although the overall mechanisms are not fully elucidated, this bacterium reinforced the gut barrier function by increasing the production of specific endocannabinoids with anti-inflammatory properties, Reg3γ, and increased the mucus layer thickness( Reference Everard, Belzer and Geurts 120 , Reference Shin, Lee and Lee 121 ). Taken together, a variety of rodent model studies indicate that probiotics may elicit beneficial impacts in metabolic disorders associated with obesity and diabetes. Moreover, several studies indicate that these effects may be exerted by a postulated role of probiotics in modulation of the intestinal barrier function, albeit via unknown molecular mechanisms. Importantly, very few studies have evaluated whether similar effects can be achieved in human studies, marking a major caveat in our understanding of the possibilities of probiotic benefits in human obesity and diabetes.
Necrotising enterocolitis
NEC is an acute inflammatory process in the immature intestine, which affects 5–10 % of very low birth weight infants and results in high mortality, heavy complications and invalidating sequelae( Reference Neu and Walker 122 ). Several randomised clinical trials indicate a protective effect of probiotics in the prevention on NEC. Despite recent critical reviews and meta-analyses( Reference Ganguli and Walker 123 – Reference Mihatsch, Braegger and Decsi 127 ) that support significant reduction in the incidence of severe NEC and mortality in preterm infants by probiotics, medical experts hesitate to recommend the routine use of a specific probiotic treatment and await large and well-designed safety and efficacy trials to complement the currently available pooled analysis of relatively small trials that use different probiotic strains( Reference Mihatsch 126 , Reference Mihatsch, Braegger and Decsi 127 ). Nevertheless, most experts agree on the potential of some probiotic strains to reduce the incidence and severity of NEC. In previous papers, it has been extensively discussed that immaturity of the intestinal barrier is amongst the major aetiological factors of NEC( Reference Anand, Leaphart and Mollen 128 – Reference Sharma and Tepas 134 ) and may be modulated by probiotic administration.
Most probiotic trials in preterm infants have focused on the impact on intestinal colonisation as measured by faecal microbiota composition analyses( Reference Agarwal, Sharma and Chaudhry 135 – Reference Underwood, Salzman and Bennett 142 ). These studies have illustrated that a variety of (mixtures of) probiotic strains or synbiotics can reduce the levels of potential pathogenic bacteria and yeast species in the faecal microbiota, which could contribute to the reduction of incidence and severity of NEC. An alternative scenario proposed that some probiotic strains such as L. reuteri ATCC 55730 could play a role in accelerating the gastrointestinal motility( Reference Indrio, Riezzo and Raimondi 143 ) thereby preventing intestinal overgrowth, which is a proposed NEC risk factor.
Only two clinical trials that used the probiotic strain Bifidobacterium lactis CNCM I-3446 have addressed other components of the preterm barrier function. One of these studies demonstrated that this probiotic induced a faster decrease of intestinal permeability levels and, not surprisingly, an increased relative abundance of faecal bifidobacteria( Reference Stratiki, Costalos and Sevastiadou 144 ). Although this small study was not powered to assess the impact on NEC, three infants developed the disease within the placebo group, whereas none developed NEC in the probiotic group. In a separate study that used the same probiotic, the expected bifidogenic effect was confirmed and was accompanied by reduced counts in faecal samples of potentially pathogenic bacteria (e.g. clostridia and enterobacteria( Reference Mohan, Koebnick and Schildt 145 )), which may be related to accompanying increased faecal SCFA levels and lower faecal pH( Reference Lee, Drongowski and Coran 150 ). Notably, this study also reported decreased calprotectin (marker for intestinal inflammation) and increased serum IgA levels in faecal samples( Reference Mohan, Koebnick and Schildt 146 ), supporting the proposed impact of this probiotic on barrier function either directly or via the decreased relative abundance of pathogenic bacterial groups.
Most studies that support a role of probiotics in the acceleration of appropriate barrier function establishment in the immature intestinal tract have been generated in neonatal animal models using immature pigs, rabbits or rats to mimic the preterm infant barrier and the NEC-associated inflammatory conditions. The available data obtained in such models support that some probiotic strains can reduce the incidence and/or severity of NEC by modulating diverse components of the intestinal barrier. These studies report favourable effects on the diversity of the commensal microbiota( Reference Fak, Ahrne and Linderoth 147 – Reference Siggers, Siggers and Boye 149 ), including reduced luminal and mucosal colonisation, as well as translocation to extra-intestinal organs of pathogenic bacteria( Reference Lee, Drongowski and Coran 150 – Reference McVay, Boneti and Habib 152 ). Barrier integrity improvements measured in these models include a decreased permeability to small solutes( Reference Gareau, Jury and MacQueen 153 ), macromolecules( Reference Fak, Ahrne and Linderoth 147 , Reference Gareau, Jury and MacQueen 153 – Reference Terpend, Blaton and Candalh 156 ) and endotoxin( Reference Caplan, Miller-Catchpole and Kaup 157 ). Moreover, in these models, the mucosal expression of various factors involved in barrier function regulation and maintenance could be normalised by probiotic supplementation, including TLR4( Reference Liu, Fatheree and Mangalat 158 ) and TLR9( Reference Good, Sodhi and Ozolek 159 ), mucin and trefoil factor (TFF)( Reference Khailova, Dvorak and Arganbright 160 ) and antimicrobial protein and peptides( Reference Underwood, Kananurak and Coursodon 161 ). Probiotics were also shown to enhance enterocyte proliferation and migration( Reference Preidis, Saulnier and Blutt 148 ), to reduce apoptosis and promote epithelial cytoprotective responses( Reference Khailova, Dvorak and Arganbright 160 , Reference Lin, Nasr and Berardinelli 162 , Reference Hunter, Williams and Petrosyan 163 ), to improve the expression of the enterocyte injury markers intestinal-type and liver-type fatty-acid-binding proteins (I-FABP and L-FABP)( Reference Goncalves, Soares and Figueira 164 ), to restore the expression and localisation of TJ and adherens junction proteins( Reference Khailova, Dvorak and Arganbright 160 ) and to restore the thickness of the intestinal mucosal and muscle layers( Reference Goncalves, Soares and Figueira 164 ). Most of these trials do not inform about the specificity of the probiotic preparation related to their gut barrier effects, as they focused on the effect of one single probiotic or a probiotic mixture. However, when phylogenetically close strains were compared, they generally differentially modulated both intestinal inflammation and barrier outcomes( Reference Preidis, Saulnier and Blutt 148 , Reference Liu, Fatheree and Mangalat 158 , Reference Liu, Fatheree, Mangalat and Rhoads 165 ).
In line with this, a trial in preterm piglets fed a probiotic blend (L. paracasei ATCC55544, B. animalis DSM15954 and Streptococcus thermophilus DSM15957) either alive or inactivated by γ-irradiation reported not a decrease but an increase in the rate of NEC in the probiotic-treated animals. This was associated with impaired paracellular permeability in the group fed dead probiotics, and with exacerbated expression of proinflammatory cytokines and reduced microbiota diversity in the group fed the live probiotic blend( Reference Cilieborg, Thymann and Siggers 166 ). To the best of our knowledge, similar results have never been reported in preterm infants and, although cases of sepsis due to probiotic administration have been occasionally described in preterm( Reference Guenther, Straube and Pfister 167 ) and immunocompromised patients( Reference Land, Rouster-Stevens and Woods 168 ), these cases remain rare. As discussed by the authors, the results in the piglet study may be explained by experimental bias and would require confirmation( Reference Cilieborg, Thymann and Siggers 166 ). Nevertheless, this study fuels safety concerns of probiotic applications in the highly sensitive preterm infant population, and supports the request for larger-scale safety and efficacy studies mentioned above.
Taken together, the evidence in neonatal animal models supports that probiotics can strengthen a variety of the immature gut barrier components, which can contribute to reduced incidence and/or severity of NEC. However, only few human studies evaluated barrier function modulation by probiotics in preterm infants and largely focused on their impact on microbial colonisation and microbiota composition. Most of these studies reported reduced pathogen loads in the developing preterm intestine upon probiotic administration, and some of them accelerated maturation of specific barrier components. The preclinical data suggest probiotic specificity on their barrier modulatory activity and therefore the need for a careful evaluation of each probiotic preparation.
Overall, current knowledge could translate into recommended routine use of specific probiotics in preterm infants, provided that large-scale and well-designed multicentre validation studies confirm their efficacy and safety.
Irritable bowel syndrome
IBS is a gastrointestinal disorder characterised by the association between recurrent abdominal pain/discomfort and a change in stool consistency or frequency. IBS has been classified according to dominant symptomatology in subgroups as constipation-predominant, diarrohea-predominant and mixed-type IBS. The worldwide prevalence is estimated to be 10–20 %, thereby causing an important health and social burden with substantial medical costs( Reference Levy, Olden and Naliboff 169 , Reference Koloski, Talley and Boyce 170 ). Increased visceral perception and deregulated mucosal immune responses are characteristics of IBS directly related to deterioration of intestinal barrier function. After an initial report from Finland( Reference Kassinen, Krogius-Kurikka and Makivuokko 171 ), increasing evidence emerged on distinct and various alterations in gut microbial composition in subgroups of IBS patients around the globe( Reference Kassinen, Krogius-Kurikka and Makivuokko 171 – Reference Jeffery, O’Toole and Ohman 175 ). Separate analysis of the faecal and mucosa-associated microbiome in IBS revealed specific patterns and correlates with mucosal lymphocyte phenotypes( Reference Sundin, Rangel and Fuentes 176 ). Although the scientific debate is ongoing, this suggests that at least in subpopulations of the IBS patient pool the gut microbiota is likely contributing to disease symptoms through an altered fermentation process, a harmful modulation of enteric sensorimotor function, an impaired intestinal barrier function, sustained immune activation without macroscopic signs of inflammation and a harmful modulation of the brain–gut axis( Reference Tojo, Suarez and Ruas 177 – Reference Hyland, Quigley and Brint 180 ). Moreover, specific probiotics have demonstrated effects on barrier function( Reference Karczewski, Troost and Konings 25 ), suggesting these bacteria might have a beneficial impact on IBS symptom scores. Mostly, in vitro and ex vivo cell culture( Reference Nebot-Vivinus, Harkat and Bzioueche 181 , Reference Laval, Martin and Natividad 182 ), as well as animal models such as mice( Reference Nebot-Vivinus, Harkat and Bzioueche 181 – Reference Wang, Gong and Wang 183 ) and rats( Reference Da Silva, Robbe-Masselot and it-Belgnaoui 184 , Reference Agostini, Goubern and Tondereau 185 ), have been used in which epithelial barrier integrity is experimentally disrupted before the addition of (mixtures of) a probiotic strain. Several of these studies demonstrated alleviation of IBS-like symptoms in animal models and importantly also showed restoration of barrier function, which was likely due to the enhanced expression of TJ proteins such as occludin, E-cadherin and claudin-1 observed in the probiotic-treated groups. Probiotics could be a promising treatment option in IBS, and several, often small-scale, randomised clinical trials have been performed with various strains or mixtures of strains. Overall, the results of these trials are difficult to interpret, because of the use of different strains and combinations thereof, as well as limitations in the study designs( Reference Koenig and Brummer 186 ). Nevertheless, the current consensus in the scientific community is that there is a beneficial potential of the administration of specific probiotics strains in IBS, but that more robust clinical trials are required( Reference Whelan 187 – Reference Gibson, Brummer and Isolauri 190 ). Particularly, lactic acid bacteria have been selected as promising probiotic candidates in the treatment of IBS( Reference Clarke, Cryan and Dinan 191 ). Although the concept seems attractive, it is not well established that a multi-strain or multi-species probiotic therapy would be preferable to single-strain administration. Irrespective of the outcome of these trials, it remains to be established whether eventual beneficial effects of probiotic supplementation in IBS are mediated through effects on barrier function.
A further area that deserves attention is the combination of selected non-digestible polysaccharides and other prebiotic compounds with specific probiotic strains (denoted as synbiotics), which could favour the production of lactic acid and butyrate. Such approaches may stimulate synergistic effects of probiotics and the endogenous microbiota and their metabolites, and could provide new scenarios to generate beneficial health effects in IBS patients. This is of particular interest in the light of the significant differences that have been reported for the intestinal microbiota composition when comparing IBS patients and healthy controls in both adult( Reference Rajilic-Stojanovic, Biagi and Heilig 172 ) and children( Reference Saulnier, Riehle and Mistretta 192 ) cohorts. The role of butyrate is especially interesting in this perspective, because it has been shown to alleviate the perception of afferent visceral signalling and, as a consequence, could improve IBS symptoms( Reference Vanhoutvin, Troost and Kilkens 193 ).
More research is also required to improve the colonisation of probiotic bacteria, as well as to identify optimal duration and possibly interruption of probiotic therapy in IBS. Moreover, further work is needed to better understand the possible interplay between probiotics and the endogenous microbiota, which could affect IBS via various mechanistic scenarios. Another important step forward would be targeting of carefully characterised subpopulations on the basis of pathophysiology rather than symptomatology, enabling a more personalised approach in the future. In probiotic studies that target the IBS population, a careful characterisation of alterations along the microbe–gut–brain axis is highly recommended and may support eventual probiotic therapies in IBS. Inversely, in view of the importance of the microbe–gut–brain axis in IBS, it is plausible that therapeutic option may also be found in the improvement of certain aspects of brain function, including mood, stress response and processing, with the aim to improve symptoms in IBS patients, possibly by altering microbial composition and/or activity in the intestine.
Inflammatory bowel disease
IBD is the overall term used to describe 2 idiopathic inflammatory diseases of the gut, namely Crohn’s disease (CD) and ulcerative colitis (UC). These diseases, however, are quite different. CD can affect any part of the bowel but predominantly the terminal ileum and colon and is characterised by granulomatous, patchy, transmural mononuclear inflammation. In contrast, UC only affects the colon and the lesions are mucosal and continuous, with massive neutrophil infiltration, crypt abscesses, loss of goblet cells and epithelial cell damage. There is rather strong evidence that the immune response in CD is directed against the indigenous microbiota, but in UC the role of the microbiota is more contentious( Reference MacDonald, Monteleone and Fantini 194 ). In active IBD, mucosal inflammation is accompanied by impaired barrier function( Reference Pastorelli, De Salvo and Mercado 195 ). The effect of inflammation on the barrier is likely to have many components. Pro-inflammatory cytokines such as interferon-γ ( Reference Beaurepaire, Smyth and McKay 196 ) and TNF-α make the epithelium leaky( Reference Gibson 197 ). Cytokine IL-13 induces up-regulation of claudin 2, changing ion transport across the epithelium( Reference Prasad, Mingrino and Kaukinen 198 ). Matrix metalloproteinases such as matrix metallopeptidase (MMP)-12 made by activated macrophages and MMP-3 made by activated myofibroblasts degrade the epithelial basement membrane and disrupt epithelial cell adhesion leading to anoikis( Reference Biancheri, Di Sabatino and Corazza 199 ). Free radicals and serine proteinases made by neutrophils directly kill epithelial cells.
Many studies have evaluated the potential of probiotics to contribute to IBD treatment. However, a fundamental problem with these studies is that different probiotics were used, either alone or in combination with other therapies. This makes the case that probiotics might work in IBD, but so far, we have not discovered the correct organism or combination of organisms. One should also note that IBD diseases are very serious conditions. For example, the induction of remission in CD requires powerful immunosuppressive agents such as corticosteroids, azathioprine and anti-TNF. In UC, the mainstay of remission induction in active disease is also corticosteroids. In view of the seriousness of these diseases, the idea that probiotics would be useful in treating active IBD is rather fanciful. Instead, there is much more of an opportunity to determine whether probiotics can help in maintaining remission, where their effects on maintaining the barrier are more relevant.
Three studies (with largely the same protocol) have investigated whether probiotic therapy prevents endoscopic recurrence of CD after surgical resection. It is very well established that recurrence of disease is first seen proximal to the anastomosis after resection. Although different probiotics were used in each study, the conclusions reached in each were consistent, indicating that no advantage was observed in the probiotic-treated groups compared with the placebo controls( Reference Prantera, Scribano and Falasco 200 – Reference Van Gossum, Dewit and Louis 202 ). Synbiotic therapies combine probiotics with prebiotics, and although an initial synbiotic study did not report any benefit in post-operative occurrence of CD( Reference Chermesh, Tamir and Reshef 203 ), a more recent study reported a slight but significant enhancement of the induction of remission of active CD( Reference Steed, Macfarlane and Blackett 204 ). Taken together, the use of probiotics as a therapy for active CD or for maintenance of disease remission has been disappointing, which has also been concluded in various recent reviews( Reference Ghouri, Richards and Rahimi 205 , Reference Whelan and Quigley 206 ). Notably, more promising results have been obtained for the use of probiotics in therapeutic applications to induce remission of UC, but also for supporting the maintenance of UC remission( Reference Ghouri, Richards and Rahimi 205 ). In a double-blind study comparing mesalazine with an oral preparation of viable E. coli strain Nissle (Serotype 06: K5: H1) with regard to their efficacy in preventing a relapse of the disease over a period of 12 weeks, probiotic treatment was equivalent to mesalazine( Reference Kruis, Schutz and Fric 207 ).
Pouchitis is an iatrogenic condition that occurs when a pouch is formed from terminal ileum and anastomosed onto the rectal stump following colectomy for UC, giving patients the opportunity to live without a stoma. Most patients develop some form of inflammation in the neo-reservoir (pouchitis). Standard therapy is the use of antibiotics such as metronidazole and ciprofloxacin. A number of studies in children and adults have demonstrated that taking a mixture of probiotic strains (VSL#3) is effective in preventing pouchitis and also can play a significant role in treating active pouchitis( Reference Ghouri, Richards and Rahimi 205 ). It is likely that the efficacy of this probiotic preparation in pouchitis is due to the highly unusual situation in the pouch that forms a reservoir for ileal fluid, which could create an environment that allows probiotics to competitively inhibit potential harmful bacteria and fungi and thereby have its positive effects in pouchitis.
In terms of probiotic effects on the compromised epithelial barrier function in IBD, they may be exerted by different principal modes of action. First, probiotics may dampen mucosal inflammation activity, thereby allowing the epithelial function to return to normal. One intensively studied way to achieve suppression of mucosal inflammation is through the modulation of the balance and activity of the mucosal T-cell repertoire, particularly focused on stimulation of the regulatory T-cell populations( Reference Veerappan, Betteridge and Young 208 ). Second, and more important in the context of this review, probiotics may directly affect the epithelial cells, leading to restoration of mucosal barrier integrity, thereby lowering the luminal antigenic stimulation that drives inflammation and suppressing inflammatory responses.
Many in vitro studies have demonstrated that probiotics can maintain the epithelial barrier and protect against cytokine-driven epithelial barrier dysfunction. Work by Polk et al. has taken this to a new level by isolating a P40 component of L. rhamnosus GG, which prevents cytokine-induced epithelial barrier dysfunction by signalling through the epidermal growth factor receptor( Reference Yan, Cao and Cover 209 ). When formulated for delivery into the mouse gut, P40 ameliorates colitis induced by DSS. In the same mouse model, a variety of studies highlights the potential of single strain or mixtures of probiotics to thicken the mucus layer and/or improve epithelial barrier function in general, with beneficial consequences for the inflammation read-outs in these animals( Reference Toumi, Abdelouhab and Rafa 210 – Reference Ahl, Liu and Schreiber 213 ). A soluble factor from Bifidobacterium infantis also increases occludin and ZO-1 in epithelial cells in vitro and ameliorates colitis in IL-10 knock-out mice( Reference Ewaschuk, Diaz and Meddings 214 , Reference Yan, Liu and Dempsey 215 ). These data have also been taken into humans where it has been shown that L. plantarum perfusion in the duodenum, elicits structural changes in ZO-1 and occludin complexes that correlate with enhanced barrier function( Reference Karczewski, Troost and Konings 25 ). These examples do not provide a complete view of the available data, but there seems to be little doubt that probiotics and probiotic components can influence epithelial barrier integrity, and further molecular characterisation may resolve how specific probiotic components can affect the receptors and pathways they modulate. These insights could reinforce the specific role of probiotics in the treatment of UC and pouchitis, but is unlikely to change the perspective on effective probiotic treatment or maintenance of remission in CD.
Concluding remarks
Intestinal barrier integrity is a hallmark of gut health and a reflection of appropriate microbiota-accommodating homeostasis of mucosal function, which is orchestrated to combine maximised absorptive capacity with appropriate defensive reactions against chemical and microbial challenges. Many factors contribute to mucosal barrier function, including epithelial integrity, which is protected by the mucus layer that creates a physicochemical barrier that prevents direct contact of luminal challenges with the epithelia and is further supported by innate and adaptive immune functions. The endogenous microbiota play a key role in establishing mucosal barrier function development in infancy, but also in maintenance of this function during later life( Reference Rescigno 216 ).
The production of butyrate by the fermentative activity of the microbiota has been reported to increase production of secreted mucus and support regulatory T-cell functions in the gut( Reference Atarashi, Tanoue and Shima 217 – Reference Furusawa, Obata and Fukuda 219 ). Thus, modulation of the butyrate-producing sub-consortia of microbiota through prebiotics or dietary fibre supports the idea that nutritional strategies might also be effective in enhancing epithelial barrier function.
A striking example of how microbiota modulation can have a therapeutic effect is the use of faecal transplantation (bacteriotherapy) to treat C. difficile-associated disease, which has been successful even after the failure of multiple courses of antibiotics( Reference Khoruts, Dicksved and Jansson 220 , Reference Borody and Khoruts 221 ). With the growing success of faecal transplantation for C. difficile, patients suffering from other intestinal diseases such as IBD or the metabolic syndrome have been enrolled for faecal transplantation. Indeed, recent studies on UC( Reference Borody, Warren and Leis 222 ) and the metabolic syndrome( Reference Vrieze, Van Nood and Holleman 223 ) indicated that gut microbiota infusion improved remission period length or insulin resistance, respectively, supporting the efficiency of gut microbiota transfer to improve some microbiota-related pathologies. However, studies on long-term efficiency of such microbiota transfer to elicit sustained health benefits still need to be addressed. Notably, the safety of the faecal material that is used for transplantation cannot be entirely assessed, because of the complexity of the microbial community. Consequently, there is an increasing interest in strategies aiming to define a well-characterised microbial cocktail of selected intestinal microbes that can effectively deliver the same health-promoting functionality as faecal donations, and replace faecal transplantation.
A compromised intestinal barrier is associated with several human gut-related disorders, for example IBD, NEC and low-grade inflammation associated with the metabolic syndrome (obesity and diabetes). In addition, several other diseases also appear to be associated with a ‘leaky’ gut phenotype, including the post-infectious subtype of IBS( Reference Spiller, Jenkins and Thornley 224 ), and possibly also allergy( Reference Panzer and Lynch 225 ). Several lines of evidence indicate that probiotics can influence various aspects of intestinal barrier function (see above), which would support their therapeutic potential in diseases involving a dysfunctional gut barrier. However, most of the probiotic barrier effects were studied in in vitro cell line and animal models and the translation of these results to human efficacy in vivo in at risk or diseased populations remain virtually unexplored. Only very few studies using probiotics have directly evaluated mucosal barrier function in human volunteers, and in the studies where this parameter was measured healthy volunteers rather than diseased populations were typically targeted( Reference Karczewski, Troost and Konings 25 , Reference Gotteland, Cruchet and Verbeke 26 ). Notably, both positive( Reference Stratiki, Costalos and Sevastiadou 144 , Reference Rosenfeldt, Benfeldt and Valerius 226 ) and negative( Reference Gotteland, Cruchet and Verbeke 26 , Reference McNaught, Woodcock and Anderson 227 ) impacts of probiotic consumption on barrier function have been reported, suggesting that a balanced view is required to evaluate their capacity to modulate intestinal barrier function in humans, particularly when severely compromised patient groups are targeted. Importantly, in the attempt to translate the results obtained in in vitro studies and experiments that use inbred animals, one should take into account that (healthy as well as diseased) humans are highly individual. Notably, it is commonly seen that molecular responses elicited by dietary interventions in humans are small in comparison with the differences observed between participating individuals per se. This level of individuality has been captured in the ‘bandwidth of health’ concept, which may explain the unpredictable distributions of responders and non-responders in nutritional intervention trials( Reference Bron, van Baarlen and Kleerebezem 3 , Reference Klaenhammer, Kleerebezem and Kopp 228 ). Therefore, high-resolution, molecular phenotyping of dietary trial participants may offer new avenues to select subgroups of individuals predicted to be more responsive to the molecular response elicited by the probiotic treatment, for example, are predicted to benefit from a specific mechanism of barrier function improvement. These avenues would evolve to a more personalised nutrition approach and abandon the ‘one size fits all’ concept that is used in most studies to date.
Despite the difficulties in translating in vitro and animal study outcomes to human populations, a variety of studies imply that mucosal barrier function can be improved by probiotic treatment. This starting point underpins the need for studies in well-diagnosed diseased populations (e.g. including high resolution molecular profiling) where this health parameter is directly assessed in a probiotic intervention regimen. The current literature does not report this type of human studies, which may provide pivotal scientific support for the health-promoting therapeutic potential of probiotic treatment in disease, with a plausible underlying mode of action.
Acknowledgements
This work was conducted by an expert group of the European branch of the International Life Sciences Institute (ILSI Europe). The authors thank Dr Arthur Ouwehand who is a member of the Probiotics Task Force for his active contribution to this work.
The expert group received funding from the ILSI Europe Probiotic Task Force. Industry members of this task force are listed on the ILSI Europe website at www.ilsi.eu. For further information about ILSI Europe, please email [email protected] or call +32 2 771 00 14. The opinions expressed herein and the conclusions of this publication are those of the authors and do not necessarily represent the views of ILSI Europe, nor those of its member companies. P. D. C. is a research associate at FRS-FNRS (Fonds de la Recherche Scientifique), and P. D. C. is the recipient of grants from FNRS and the French Cancer Research Association (ARC). This work was supported by the Fonds de la Recherche Scientifique – FNRS for the FRFS-WELBIO under grant no. WELBIO-CR-2012S-02R. This work is supported in part by the Funds InBev-Baillet Latour (Grant for Medical Research 2015). P. D. C. is a recipient of an ERC Starting Grant 2013 (European Research Council, Starting grant no. 336452-ENIGMO).
P. A. B. and M. K. had responsibility for producing the final version of the article. This publication was coordinated by Dr Jackie Whyte and Dr Tobias Recker, Scientific Project Managers at ILSI Europe. All authors contributed to discussions and had input into writing the article.
All authors have completed and submitted the ICMJE Form for disclosure of potential conflicts of interest.