Among B vitamins, vitamin B12 occupies a very special niche. This vitamin is produced only by bacteria and archaebacteria if Co supply is adequate. As opposed to other B vitamins, it is neither synthesised nor used by fungi and plants(Reference Martens, Barg and Warren1). Therefore, in human diets, the sole natural source of vitamin B12 comes from animal products. Among animal products, those from ruminants are particularly rich in vitamin B12, the vitamin being naturally synthesised by ruminal microflora using Co as an essential precursor and then absorbed and stored in the liver and muscles (meat) of the host or secreted in its milk(Reference Combs2).
In humans, vitamin B12 deficiency affects cell division and may lead to megaloblastic anaemia and neuropathy(Reference Combs2). In the presence of vitamin B12 deficiency, increasing folic acid supply cures anaemia but not neurological symptoms, then, by masking haematological symptoms, it could delay the diagnosis of vitamin B12 deficiency until neurological damages are irreversible. Consequently, over the last decade, since folic acid fortification of flour became mandatory in many Western countries, including Canada and USA, there has been a renewed interest in the evaluation of vitamin B12 status in human populations according to folic acid provision(Reference Selhub, Morris and Jacques3, Reference Selhub, Morris and Jacques4).
Vitamin B12 status is correlated with vitamin B12 intake in humans(Reference Tucker, Rich and Rosenberg5–Reference Bor, von Castel-Roberts and Kauwell7). Vegetarians had lower vitamin B12 status than omnivores(Reference Bor, von Castel-Roberts and Kauwell7, Reference Miller, Specker and Ho8). However, dietary sources of the vitamin also seem to matter. For example, vitamin status of vegetarians was positively correlated with their intake of dairy products, especially milk, but not of eggs or seafood(Reference Miller, Specker and Ho8). Among adults not using vitamin supplements, the relationship between plasma concentration of the vitamin and its intake from dairy products is similar to the relationship observed with the intake from cereals fortified with vitamin B12. However, the relationship with intake from meat, poultry or fish is weaker(Reference Tucker, Rich and Rosenberg5). A Norwegian study showed that plasma vitamin B12 increases with the amounts of vitamin B12 provided by dairy products or fish but not with those provided by eggs or meat(Reference Vogiatzoglou, Smith and Nurk6). Moreover, for a similar intake, plasma concentrations of vitamin B12 were higher when the vitamin was supplied by dairy products than by fish, suggesting that the bioavailability of the vitamin from dairy products is higher than from the other sources(Reference Vogiatzoglou, Smith and Nurk6). Indeed, these retrospective studies seem to indicate that vitamin B12 supplied by dairy products is more available than from other natural sources, although in these studies, intake data were obtained by a FFQ.
In terms of the provision of vitamin B12, one glass (250 ml) of milk provides more than 1 μg of vitamin B12(9). According to the Canadian Food Inspection Agency(10), cows' milk could claim to be an ‘excellent source’ of vitamin B12, because one glass of milk (250 ml) provides nearly 50 % of the RDA(11) for adults and children over 13 years of age (2·4 μg/d). Moreover, vitamin B12 supplements given to dairy cows via weekly injections increased vitamin B12 content in milk by 50 %(Reference Preynat, Lapierre and Thivierge12); in such a case, a glass of milk may provide up to 75 % of the RDA.
Cyanocobalamin is the synthetic form of vitamin B12 present in most supplements, the cyanide group being used to stabilise the molecule. However, cyanocobalamin is not biologically active until the cyanide group is enzymatically removed(Reference Herbert13). Bioavailability of the synthetic form of vitamin B12 is reported to be poor ( < 4 %) in humans and animals(Reference Zittoun14). In milk, vitamin B12 is present as adenosylcobalamin, hydroxocobalamin and methylcobalamin(Reference Farquharson and Adams15, Reference Fie, Zee and Amiot16). Hydroxocobalamin is the product of photolysis of light-sensitive cobalamins, whereas adenosylcobalamin and methylcobalamin have a coenzymatic activity in mammal cells and are biologically active(Reference Farquharson and Adams15).
It was therefore hypothesised that the important daily provision of vitamin B12 brought by unprocessed (raw) or processed milk (pasteurised or microfiltrated) is more efficiently absorbed than the synthetic form, cyanocobalamin, used in vitamin supplements. The present study aimed to compare in pigs, used as an animal model for humans, the net flux of vitamin B12 across portal-drained viscera (PDV) after ingestion of conventional and vitamin B12-enriched (via injections to cows) milk (raw, pasteurised or microfiltrated) to the equivalent amount of cyanocobalamin or to an unsupplemented control diet.
Experimental methods
Milk collection and preparation
We collected two types of milk differing in their vitamin B12 content from the dairy herd of Agriculture and Agri-Food Canada (AAFC) at Sherbrooke. Conventional milk (C; 980 l) was obtained from cows not supplemented with vitamin B12; it contained 12·0, 3·2 and 3·5 % of total solids, crude protein and fat, respectively. Vitamin B12-enriched milk (E; 820 l) was obtained at the same time from a group of eighteen cows that had received weekly intramuscular injections of 2 ml of a solution of cyanocobalamin (5 mg/ml) during three consecutive weeks as previously employed by Girard & Matte(Reference Girard and Matte17). Vitamin B12-enriched milk composition was 11·7, 3·2 and 3·3 % of total solids, crude protein and fat, respectively. The milk was transported for processing at the AAFC Food Research and Development Centre of St Hyacinthe, QC, Canada. Upon arrival, skimmed milk and cream were separated, and the latter was kept apart. Skimmed milk was separated in three fractions. The first third was not treated (raw skimmed milk; R). The second third was microfiltrated (microfiltrated skimmed milk; F) using the ‘Bactocatch’ procedure (cold pasteurisation) as described by Trouvé et al. (Reference Trouvé, Maubois and Piot18) in a cross-flow microfiltration unit (Pilot Plant type MFS-7, Alfa-Laval MFSI, Tumba, Sweden) with a ceramic membrane pore size of 1·4 μm, membrane area of 1·4 m2, flow rate of 850 l/h per m2 and temperature of 35°C. The last third of the skimmed milk (pasteurised skimmed milk; P) and the whole cream were pasteurised (73°C for 16 s) using a plate heat exchanger Tetra Plex, C3/-SR type (Alfa-Laval, Tumba, Sweden). All skimmed milk was concentrated separately using a reverse osmosis system with a TFC® 3838 RO-N1 spiral membrane of 965 × 96 × 21·1 mm (area of 18·4 m2; Koch Membrane Systems, Inc., Wilmington, MA, USA) for final DM content of 25·9, 29·4 and 28·1 % for raw, pasteurised and microfiltrated conventional skimmed milk and 27·9, 28·0 and 30·4 % for raw, pasteurised and microfiltrated vitamin B12-enriched skimmed milk, respectively. Approximately 6 litres of each skimmed milk concentrate were freeze-dried and stored frozen at − 20°C. The pasteurised cream and the remaining of the different concentrates of skimmed milk were stored frozen at − 20°C in aliquots of 1 and 2 litres, respectively.
The vitamin B12 content of skimmed milk concentrates was determined in duplicate from two hydrolyses of each milk concentrate using a radioassay (Quantaphase B12, Bio-Rad Laboratories (Canada) Limited, Mississauga, ON, Canada) as described by Preynat et al. (Reference Preynat, Lapierre and Thivierge19).
Animals and surgeries
After weaning at 28 d of age, ten female Yorkshire–Landrace × Duroc piglets were fed ad libitum a conventional basal diet for post-weaned piglets (Table 1). This basal diet was supplemented with cyanocobalamin as recommended by the National Research Council(20) for this category of pigs (20 μg/kg). Average body weight at surgery was 48·7 (sem 1·0) kg. The surgery procedure has been described by Hooda et al. (Reference Hooda, Matte and Wilkinson21). Briefly, the animals were equipped with an ultrasonic flow probe (14 mm flow probe, SB-series: Transonic Systems, Inc., Ithaca, NY, USA) around the portal vein and a catheter inside the portal vein at 3·5 and 2·5 cm before its entry into the liver, respectively. Another catheter was inserted through the carotid artery up to the junction between the carotid and subclavian arteries.
Table 1 Ingredients and chemical composition of the basal post-weaning diet

* Biophos (monocalcium phosphate) is an inorganic P source providing 21 % available P, 18 % Ca and 0·21 % F.
† Supplied, per kg of feed: Mn, 52 mg; Zn, 174 mg; Fe, 275 mg; Cu, 130 mg; I, 1 mg; Se, 300 μg; vitamin A, 3008 IU; vitamin D3, 900 IU; vitamin E, 41 IU; vitamin K, 1·5 mg; thiamine, 2·0 mg; riboflavin, 3·5 mg; niacin, 20 mg; pantothenic acid, 15 mg; folic acid, 0·5 mg; pyridoxine, 2·0 mg; biotin, 50 μg; choline, 104 mg and vitamin B12, 20 μg.
The experimental procedures followed the guidelines of the Canadian Council on Animal Care(22) and were approved by the Institutional Animal Care Committee of the Dairy and Swine Research and Development Centre of Sherbrooke (Québec, Canada). All animals were cared for according to the recommended code of practice of Agriculture Canada(23).
Treatments
After surgery, the animals were penned individually (1 m × 1·8 m) and fed a single daily meal of 1·2 kg of the diet described in Table 1. At 10–15 d after surgery, when the animals had recovered full appetite and a normal growth rate, they were gradually (3–5 d) adapted to the metabolic cage (with free access to water) and to the consumption of a mixture of milk concentrate and cereals similar to milk treatments described below. The treatments were tested according to a split-plot design in which the concentration of vitamin B12 was the first plot. There were five pigs in each plot. Within each plot, each animal received the five experimental treatments according to a cross-over design. The three treatments corresponded to mixtures of concentrated milk (raw, microfiltrated and pasteurised), cream and cereals (67 % maize, 16·5 % wheat and 16·5 % soyabean meal) as described in Table 2. This was done to mimic a human breakfast. In the two remaining treatments, cereals were mixed to provide 1·2 kg of DM and 1·3 litres of water was added to obtain the same volume as the milk treatments; these meals were supplemented with (B12-S) or not supplemented with (B12-0) cyanocobalamin (V-2876, Sigma-Aldrich, St Louis, MO, USA), the synthetic form of the vitamin. Cyanocobalamin, approximately 50 μg (84 μl of a solution at 600 μg/ml) or 80 μg (135 μl of a solution at 600 μg/ml) corresponding to the average provision from C or E milk, respectively, was incorporated in a 25 g pellet made of the basal diet mixed with unsweetened apple sauce to induce a rapid consumption just before the meal. It was assumed that the sole source of vitamin B12 in the mixtures of milk and cereals came from milk because feedstuffs from plants are devoid of that vitamin(Reference Martens, Barg and Warren1). The analysed values for the cyanocobalamin solution were lower (524·2 (sem 5·9) μg/ml, n 7) than the calculated values. Thus, vitamin B12 intake for C–S and E–S treatments was 44 and 71 μg, respectively.
Table 2 Composition of the milk-based experimental meals and their provision of endogenous vitamin B12
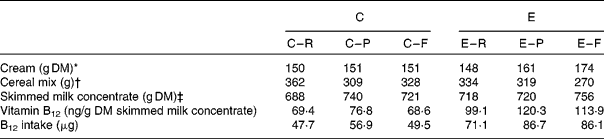
C, conventional milk; E, enriched milk; R, raw milk; P, pasteurised milk; M, microfiltrated milk.
* Amount of cream added to obtain an equivalent of 2 % fat milk.
† The mixture of skimmed milk and cream was complemented with cereals (67 % maize, 16·5 % wheat and 16·5 % soyabean meal) to provide a meal of 1·2 kg DM. The nutrient composition (analytical values) of the cereal mix was as follows: DM, 88·2; metabolisable energy, 14·1 MJ/kg; crude protein, 15·7 %; fat, 3·5 %; crude fibre, 4·1 %; Ca, 0·15 %; phosphorus, 0·54 %; ash, 3·6 %.
‡ Whole DM intake from skimmed milk concentrate in experimental meals (1·5 litres of liquid+300 g of freeze-dried).
On experimental days, the animals were placed in metabolic cages and fed one of the experimental meals. Blood samples (4 ml) were collected simultaneously from the two catheters every 45 min for the first 3 h post-feeding, and every hour for the following 21 h. Portal blood flow was recorded continuously during 24 h using the WinDaq® software (Dataq Instruments, Inc., Akron, OH, USA). Between experimental days, the animals were moved back to their pen for 3–4 d and fed the basal diet described in Table 1.
Blood collection and analysis
Immediately after sampling, the blood was transferred from syringes into EDTA-treated tubes (Vacutainer®, Becton Dickinson, Franklin Lakes, NJ, USA). Packed cell volume was measured in duplicate on fresh blood by microcentrifugation. An aliquot of blood was immediately frozen for Hb determination according to the method of Drabkin(Reference Manet24). Plasma was collected after centrifugation at 1800 g for 10 min at 4°C and frozen at − 20°C for further analysis. Plasma concentrations of vitamin B12 were measured in duplicate by radioassay in two different assays (SimulTRAC-S Radioasssay kit, Vitamin B12 (57Co)/Folate (125I), MP Biomedicals, Diagnostics Division, Orangeburg, NY, USA). Validation tests for these measurements showed satisfactory parallelism (CV = 5·0 %), and recovery tests (92·8 %, CV = 4·0 %) between 50 and 800 pg/ml. The intra- and inter-assay CV were 4·1 (n 13) and 5·6 % (n 23), respectively.
DM, crude protein, fat, crude fibre, Ca, P and ash in the conventional basal diet and in the cereal mix were analysed following AOAC(25) methods (930·15, 990·03, 920·39, 962·09, 985·01, 985·01 and 942·05, respectively).
Calculations and statistical analysis
In the C group, one pig had to be removed from the study. Signals from the flow probes were lost for the last period for one pig (treatment C–R) and for the three last periods for another pig (treatments E–P, E–F and E–S). For these two pigs, the calculations of PDV fluxes for these periods were done using the average plasma flow at each sampling time recorded during the previous periods. The estimated values for these periods were not included in statistical analysis of blood and plasma flows.
Statistical analyses of blood and plasma flows, arterial concentrations, venoarterial difference and PDV flux of vitamin B12 were conducted on postprandial values that were averaged across the 24 h post meal. The net flux of vitamin B12 across PDV was calculated as described by Girard et al. (Reference Girard, Lapierre and Desrochers26) for each sampling time. As time intervals between the blood samples were unequal, the average net flux of vitamin B12 across PDV during the 24 h post meal was calculated as the summation of PDV net flux at each time point multiplied by the interval of time between the two consecutive samples divided by 1440 min. A positive net flux indicates a release of a nutrient from PDV, whereas a negative flux indicates an uptake.
All variables were analysed using the MIXED procedure of SAS (SAS Institute Inc., Cary, NC, USA)(27) according to a split-plot design with vitamin B12 concentration and period as the main plots, treatment and the vitamin B12 concentration × treatment interaction as the subplots and the vitamin B12 concentration × period and treatment × period within vitamin B12 concentration interactions as the random effects. When the treatment effects reached a level of significance of 95 %, the following a priori comparisons were used: (1) unsupplemented v. cyanocobalamin; (2) cyanocobalamin v. all milk (raw, pasteurised and microfiltrated); (3) raw v. treated (pasteurised+microfiltrated) milk; (4) pasteurised milk v. microfiltrated milk. The results are reported as least square means and standard errors of the means. The significance level was defined at P ≤ 0·05, and trends towards significance were considered at 0·05 < P < 0·10.
Results
The pigs in the E group had lower (P = 0·04) blood and plasma flows than those in the C group (1·08 (sem 0·05) v. 1·31 (sem 0·06) and 0·75 (sem 0·04) v. 0·91 (sem 0·04) litres/min, respectively). There was no treatment or vitamin B12 concentration × treatment interaction effect (P ≥ 0·7) on these two variables.
There was no effect (P ≥ 0·3) of vitamin B12 concentration, treatment or their interaction on arterial plasma concentration of vitamin B12 (186 (sem 9) pg/ml).
Average portoarterial difference and PDV flux of vitamin B12 during the 24 h post meal were not affected by vitamin B12 concentration (P ≥ 0·5) but differed among treatments (P ≤ 0·02; Table 3). Average portoarterial difference in vitamin B12 during the 24 h post meal was higher (P = 0·001) when the pigs were fed milk (raw+pasteurised+microfiltrated) than those fed cyanocobalamin. However, there was no difference (P ≥ 0·5) between raw and treated (pasteurised+microfiltrated) milk or between pasteurised and microfiltrated milk. The portoarterial difference of vitamin B12 was not different (P = 0·8) when the pigs were fed the unsupplemented diet or cyanocobalamin supplements.
Table 3 Average portoarterial difference and portal-drained viscera (PDV) flux of vitamin B12 during the 24 h post-meal according to the treatments
(Least square means with their standard errors)

* Values for unsupplemented did not differ from those of cyanocobalamin (P = 0·76), cyanocobalamin differed from those of all milks (raw, pasteurised and microfiltrated; P = 0·001), raw milk did not differ from those of treated (pasteurised+microfiltrated) milks (P = 0·83) and pasteurised milk did not differ from those of microfiltrated milk (P = 0·45).
† Values for unsupplemented did not differ from those of cyanocobalamin (P = 0·68), cyanocobalamin differed from those of all milks (raw, pasteurised and microfiltrated) (P = 0·003), raw milk did not differ from those of treated (pasteurised+microfiltrated) milks (P = 0·83) and pasteurised milk did not differ from those of microfiltrated milk (P = 0·76).
‡ These values are not different from 0 (P>0·13).
Similarly, PDV fluxes of vitamin B12 during the 24 h post meal were not different (P = 0·68) when the pigs were fed the unsupplemented diet or cyanocobalamin supplements, and these fluxes were not different from 0 (P ≥ 0·13; Table 3). PDV flux of the vitamin was higher (P = 0·003) when the pigs were fed milk than those fed cyanocobalamin. PDV fluxes of vitamin B12 were different from 0 (P ≤ 0·05) when the pigs were fed milk, but it did not differ (P = 0·8) between raw and treated milk or between milk treatments (Table 3). The cumulative PDV fluxes for 24 h varied from 5·5 to 6·8 μg. These values correspond to an efficiency of intestinal absorption of vitamin B12 from milk variying between 8 and 10 %.
Discussion
In the present study, pigs were used as a model to estimate the bioavailability of vitamin B12 in humans. In a recent review, Guilloteau et al. (Reference Guilloteau, Zabielski and Hammon28) highlighted the numerous similarities (anatomy, physiology, digestion, absorption and metabolism) between the gastrointestinal tract of humans and pigs. More specifically for vitamin B12, the bioavailability of dietary supplements of cyanocobalamin has recently been studied(Reference Matte, Guay and Le Floc'h29) in this species using two different methods. A first one, used as a reference method, measured whole-body deposition of vitamin B12 after several days of dietary supplementation, whereas the second method measured the net flux of vitamin B12 across PDV following a single meal supplemented with cyanocobalamin. The second method requires sophisticated surgical preparation, but it has the great advantage of measuring the bioavailability of different concentrations or sources of vitamin B12 within the same animal. Although estimates of bioavailability varied according to the two methods, both showed an inverse relationship between dietary concentrations of vitamin B12 and bioavailability of this vitamin as reported previously for human subjects(Reference Herbert30). Those results are in accordance with the observations that the mechanisms of absorption of vitamin B12 in pigs (an omnivorous species) are similar to those described in human subjects(Reference Combs2, Reference Schneider and Stroiński31) supporting that the pig is an appropriate model to estimate vitamin B12 availability for human subjects.
In the present study, the mean PDV flux of vitamin B12 during the 24 h following a meal not supplemented with vitamin B12 was not different from 0, as observed previously in pigs by Matte et al. (Reference Matte, Guay and Le Floc'h29). However, in this last study, the cumulative net PDV fluxes of vitamin B12 during the 24 h following boluses of 25 and 250 μg of dietary cyanocobalamin were positive at 2·4 and 5·1 μg, respectively, and differed between dietary concentrations. In contrast, in the present study, the actual mean net PDV fluxes of vitamin B12 after ingestion of meals supplemented with 44 and 71 μg of cyanocobalamin were not different from 0. The major difference between Matte et al. (Reference Matte, Guay and Le Floc'h29) and the present study was the composition of the experimental meals. In Matte et al. (Reference Matte, Guay and Le Floc'h29), a semi-purified diet, containing 16 % of vitamin-free casein (derived from cows' milk), 67 % maize starch, 11 % sucrose and 6 % mineral and vitamin supplements, was used, whereas, in the present study, the experimental meal was based on plant-derived feedstuffs (maize, wheat and soyabean meal). It can be hypothesised that a milk component, which survived the technological process for production of vitamin-free casein, improves intestinal absorption of vitamin B12. In fact, casein itself could be involved because fractions of this protein were identified as major components of the protein-binding capacity of vitamin B12 in cows' milk(Reference Gizis, Kim and Brunner32). Globally, these explanations are in accordance with the numerically greater, although not statistically significant, efficiency of absorption of dietary 58Co-labelled cyanocobalamin when given in milk (65 %) rather than in water or bread (55 %)(Reference Russell, Baik and Kehayias33). Nevertheless, it cannot be ruled out that the current lack of vitamin B12 absorption after ingestion of meals supplemented with cyanocobalamin (C–S and E–S) was related to the fibre content of feedstuffs, which could interfere with the intestinal bioavailability of dietary cyanocobalamin as observed by Cullen & Oace(Reference Cullen and Oace34).
In milk treatments, the cumulative net PDV flux of vitamin B12 for 24 h varied between 5·5 and 6·8 μg and was not significantly affected within the range of dietary intakes of conventional (48–57 μg) and enriched milk (71–87 μg). It cannot be ruled out that the present experimental approach based on the quantification of the net flux of vitamin B12 through the PDV was not sensitive enough to detect the difference within this range of vitamin B12 intakes ingested in one single supplemented meal. Such PDV flux values for vitamin B12 were previously observed following ingestion of 250 μg of cyanocobalamin incorporated in a semi-purified diet containing vitamin-free casein(Reference Matte, Guay and Le Floc'h29). Enhanced PDV flux of vitamin B12 from milk could also be related to the forms of cobalamins present in milk. In milk, vitamin B12 is mostly present as adenosylcobalamin and hydroxocobalamin whereas methylcobablamin and cyanocobalamin are present in lesser amounts(Reference Farquharson and Adams15, Reference Fie, Zee and Amiot16). Rapid pasteurisation had few effects on vitamin B12 content in milk(Reference Karlin, Hours and Vallier35). To the best of our knowledge, there is no information on the fate of the different cobalamins in milk after microfiltration. Information on the relative intestinal availability of the different cobalamins is scarce. The only data available compared whole-body retention of crystalline radioactive forms of different cobalamins in human subjects(Reference Weissberg and Glass36, Reference Adams, Ross and Mervyn37). At doses between 100 and 1000 μg, Weisberg & Glass(Reference Weissberg and Glass36) observed no difference between cyanocobalamin and hydroxocobalamin. At 25 μg, the whole-body retention of vitamin B12 was higher after the ingestion of crystalline forms of adenosylcobalamin, hydroxocobalamin and methylcobalamin than cyanocobalamin(Reference Adams, Ross and Mervyn37). Therefore, along with the abovementioned possible interaction with casein, cobalamin forms naturally present in milk could also contribute to explain the increased net PDV flux of the vitamin following ingestion of milk compared with cyanocobalamin.
In conclusion, the present results, reporting that vitamin B12 naturally and abundantly present in cows' milk is also more available at the intestinal level than its synthetic form, are in accordance with retrospective studies in human subjects showing that vitamin B12 status is highly correlated with dairy product intake(Reference Tucker, Rich and Rosenberg5, Reference Vogiatzoglou, Smith and Nurk6, Reference Miller, Specker and Ho8). Therefore, cows' milk could become a unique prophylactic tool to prevent vitamin B12 deficiencies in human nutrition.
Acknowledgements
The authors are grateful to M. Guillette, G. Danyod and G. Bélanger for their invaluable technical assistance and to C. Perreault, S. Bourgault, C. Roy and D. Morrissette for their animal care assistance. None of the authors had a financial or personal conflict of interest in relation to the present study. The study was carried out with support from Dairy Farmers of Canada and the Matching Investment Initiative program of Agriculture and Agri-Food Canada. The study sponsors had no role in the design and conduct of the study; the collection, management, analysis and interpretation of the data; the preparation, approval and submission of the manuscript. J. J. M., F. G. and C. L. G. contributed to the design of the study, interpretation of the results, writing of the manuscript, revision of its content and approval of the final version submitted for publication. J. J. M. was the principal investigator and supervised data collection; C. L. G. supervised laboratory analysis and performed the statistical analysis.