Implications
Disturbances of mineral homeostasis are of significant relevance not only in dairy cows but also in beef cattle and small ruminants. In addition, the contribution of excreted phosphorus to the pollution of surface waters necessitates a revision of our livestock feeding regimes. The present review gives an overview on our current knowledge of the regulation of mineral transport across gastrointestinal and renal epithelia derived from functional and structural studies in different ruminant species as affected by age, lactation, feeding regime, etc. It highlights the physiological differences between monogastric animals and ruminants as well as the importance of combining different scientific approaches to improve our understanding of the complex mechanisms crucial for the maintenance of mineral homeostasis.
Introduction
Depending on management strategies, milk fever occurs in dairy cows with an incidence of 0% to 1%, 1.4% to 4%, and 5.7% to 6% in the first, the second and the third lactation, while the prevalence of subclinical hypocalcaemia defined as serum Ca concentration <2 mM amounts to 5.7% to 25%, 29.0% to 41%, and 49% (Reinhardt et al., Reference Reinhardt, Lippolis, McCluskey, Goff and Horst2011; Venjakob et al., Reference Venjakob, Borchardt and Heuwieser2017). The physiological response to transient hypocalcaemia is an increase in bone mobilisation followed by enhanced gastrointestinal absorption (van´t Klooster, Reference van´t Klooster1976). If these mechanisms are compromised, either the extent or the duration of hypocalcaemia is exacerbated resulting in increased risks of developing different diseases in early lactation depending on the duration of hypocalcaemia (Neves et al., Reference Neves, Leno, Bach and McArt2018). Reliable data on the prevalence of peripartum hypocalcaemia in small ruminants are scarce. Like cows, goats develop hypocalcaemia usually at the onset of lactation, while Ca homeostasis of sheep is generally more severely challenged during late gestation (Oetzel, Reference Oetzel1988; Brozos et al., Reference Brozos, Mavrogianni and Fthenakis2011).
Homeostatic control of phosphate (Pi) is also challenged at the onset of lactation. Subclinical hypophosphatemia around parturition is observed in >50% of dairy cows (Macrae et al., Reference Macrae, Whitaker, Burrough, Dowell and Kelly2006), and low serum Pi concentrations in cows suffering from milk fever are associated with an increased risk of developing downer cow syndrome (Menard and Thompson, Reference Menard and Thompson2007). However, high P percentage of pre-calving diets was identified as a risk factor for hypocalcaemia in a meta-analysis (Lean et al., Reference Lean, DeGaris, McNeil and Block2006), and a prepartum ration low in P seems to have beneficial effects on Ca homeostasis, probably because of an impact on bone mobilisation and vitamin D metabolism (Cohrs et al., Reference Cohrs, Wilkens and Grunberg2018).
Restriction of P and CP intake may occur for economic reasons or because animals are kept on deficient pastures (McGrath et al., Reference McGrath, Savage, Nolan and Elliott2012; Elfers et al., Reference Elfers, Wilkens, Breves and Muscher-Banse2015). This might be especially relevant in growing or fattening animals. On the other hand, environmental pollution with Pi and N of animal origin is leading to legal incentives to reduce the P and CP content of ruminant rations to the lowest possible level that does not negatively affect health and productivity.
In contrast to monogastric species, including rats and horses, no significant changes in renal Ca and Pi excretion are observed in bovines kept on restricted alimentary Ca supply, and the adaptation of gastrointestinal absorption seems to be less pronounced (Martz et al., Reference Martz, Belo, Weiss and Belyea1999; van Doorn et al., Reference van Doorn, van der Spek, Everts, Wouterse and Beynen2004; Zhang et al., Reference Zhang, Lai, Leung, Che and Wong2008; Taylor et al., Reference Taylor, Knowlton, McGilliard, Swecker, Ferguson, Wu and Hanigan2009). In Table 1 we present data from balance studies carried out in different ruminant species to illustrate that Ca and P absorption and secretion out of and into different gastrointestinal segments as well as urinary excretion are influenced by age, lactation and type of diet. There is inconsistency in the contribution of the forestomach of ruminants to overall Ca absorption (Table 1), which can partly be explained with differences in the composition of rations as mineral homeostasis interferes with other dietary factors such as dietary cation–anion difference, Mg and CP supply (Goff, Reference Goff2008; Muscher and Huber, Reference Muscher and Huber2010; Elfers et al., Reference Elfers, Liesegang, Wilkens, Breves and Muscher-Banse2016a; Wilkens et al., Reference Wilkens, Elfers, Schmicke, Breves and Muscher-Banse2018). Therefore, studies using a more mechanistic approach are an important tool to enhance our knowledge.
Table 1 Results from balance studies done with different ruminant species: intake, urinary excretion (UEX), pre-intestinal (PRE) and intestinal (INT) net absorption (ABS), faecal excretion (FEX) in grams per day, apparent digestibility (AD) in percentage

GS = ryegrass silage; F = formic acid; FF = formic acid and formaldehyde; SM = soybean meal; GCS = ryegrass and clover silage; DCAD = dietary cation–anion difference; 25-OHD = 25-hydroxyvitamin; n.d. = not determined.
Unfortunately, most research on the physiological mechanisms to maintain mineral homeostasis has used rodents as models for mammals in general. It is, therefore, the aim of this review to summarise the most important peculiarities of Ca and Pi transport across gastrointestinal and renal epithelia found in ruminants and highlight differences in comparison to monogastric animals. Throughout the following text, specific results obtained in ruminants will be indicated, while more general aspects often refer to studies done in rats and mice.
Methods
To evaluate renal and gastrointestinal Ca or Pi absorption and secretion in vivo, several different quantitative methods applied: balance studies using intact or cannulated animals, radioisotope tracer techniques, and the administration of stable strontium that can be used for this purpose, as its absorption shows a close correlation with that of Ca. As these experiments do not give explanations for sometimes inconsistent results, ex vivo methods are necessary to reveal the underlying mechanisms more precisely: isolated perfused organs, micropuncture experiments on renal transport, the everted sac technique that allows to control the composition of the luminal and serosal buffer solution and thus the chemical gradient, and the Ussing chamber is used to investigate transport mechanism by altering both the chemical and electrical gradients across the epithelium to differentiate between passive and active, paracellular and transcellular mechanisms. These functional studies are completed by in vitro experiments – for example, the quantification of RNA and protein expression of transporters and the functional characterisation applying electrophysiological techniques on cloned transporters. Although all these methods can greatly improve our understanding of physiological processes, the artificial conditions used or the fact that transporter abundance does not always represent in vivo activity may also provide challenges in interpretation. Taken together, our knowledge will probably increase if we combine the information derived from all these different approaches.
Endocrine control of calcium and phosphate transport
The concentrations of ionised Ca (Ca2+) and Pi in blood are regulated in a narrow range by 1,25-dihydroxyvitamin D3 (1,25-(OH)2D3), parathyroid hormone (PTH), calcitonin and fibroblast growth factor 23 (FGF23). Homeostasis is maintained by the interplay of gastrointestinal absorption, renal resorption and mobilisation of these inorganic ions from bone. Within minutes, a drop in blood Ca2+ induces the release of PTH from the parathyroid gland (Kumar and Thompson, Reference Kumar and Thompson2011) that stimulates the mobilisation of Ca and Pi from the skeleton (Ben-awadh et al., Reference Ben-awadh, Delgado-Calle, Tu, Kuhlenschmidt, Allen, Plotkin and Bellido2014). In monogastric animals, PTH also increases renal Ca resorption and Pi excretion by direct, rapid mechanisms (Besarab and Swanson, Reference Besarab and Swanson1982). Furthermore, PTH enhances the expression and activity of 1α-hydroxylase (CYP27B1), an enzyme that converts 25-hydroxyvitamin D3 (25-OHD3) to the biologically most active vitamin D metabolite 1,25-(OH)2D3 (Fraser and Kodicek, Reference Fraser and Kodicek1973). Furthermore, direct effects of plasma Ca and Pi on 1,25-(OH)2D3 concentrations were shown in rats (Bushinsky et al., Reference Bushinsky, Riera, Favus and Coe1985; Bushinsky et al., Reference Bushinsky, Nalbantian-Brandt and Favus1989).
In lactating animals, PTH-related peptide (PTHrP) is secreted by the mammary gland into both milk and blood. Although it can bind the PTH receptor, it is probably not involved in vitamin D metabolism but likely to act on bone mobilisation (Hernández-Castellano et al., Reference Hernández-Castellano, Hernandez and Bruckmaier2019). In addition, it was suggested to exert effects on renal Ca handling such as prolactin (Herm et al., Reference Herm, Muscher-Banse, Breves, Schröder and Wilkens2015).
Depending on the concentrations of plasma Ca and calcitonin, 1,25-(OH)2D3 either increases or inhibits bone mobilisation (Kurbel et al., Reference Kurbel, Radic, Kotromanovic, Puseljic and Kratofil2003). Via its genomic effects on Ca transporter expression that become present after a certain time lag, 1,25-(OH)2D3 stimulates renal resorption and intestinal absorption of Ca (Dusso et al., Reference Dusso, Brown and Slatopolsky2005) and limits its own synthesis by inhibiting CYP27B1 and stimulating the expression of 24-hydroxylase, the enzyme that initiates the inactivation of both 25-OHD3 and 1,25-(OH)2D3 (Chen and DeLuca, Reference Chen and DeLuca1995; Beckman and DeLuca, Reference Beckman and DeLuca2002).
In addition, 1,25-(OH)2D3 induces the production of a bone-derived phosphatonin, FGF23 (Saji et al., Reference Saji, Shigematsu, Sakaguchi, Ohya, Orita, Maeda, Ooura, Mima and Negi2010), that interacts with PTH expression and vitamin D metabolism and thus decreases plasma concentrations of 1,25-(OH)2D3 (Schiavi and Kumar, Reference Schiavi and Kumar2004; Krajisnik et al., Reference Krajisnik, Björklund, Marsell, Ljunggren, Åkerström, Jonsson, Westin and Larsson2007). Low dietary P intake decreased plasma concentrations of FGF23 and concomitantly increased 1,25-OH2D3 while plasma PTH was low (Antoniucci et al., Reference Antoniucci, Yamashita and Portale2006).
Protein intake also interferes with vitamin D metabolism. Growth hormone acts mainly through insulin-like growth factor 1 (IGF1). Uncoupling of this somatotropic axis indicated by low IGF1 plasma concentrations was observed during dietary protein restriction in growing goats and in peripartum dairy cows (Muscher et al., Reference Muscher, Piechotta, Breves and Huber2011; Piechotta et al., Reference Piechotta, Holzhausen, Araujo, Heppelmann, Sipka, Pfarrer, Schuberth and Bollwein2014). Reduced IGF1 was associated with decreased expression of CYP27B1 and affected bone mobilisation and intestinal Ca absorption probably via diminished plasma concentrations of 1,25-(OH)2D3 (Wilkens et al., Reference Wilkens, Elfers, Schmicke, Breves and Muscher-Banse2018).
As all these aspects might interfere with the strategies applied to stabilise mineral homeostasis in dairy cows and beef cattle – for example, dietary interventions, vitamin D supplementation, oral and parental administration of Ca, low IGF1 during negative energy balance, etc. (Reist et al., Reference Reist, Erdin, von Euw, Tschuemperlin, Leuenberger, Delavaud, Chilliard, Hammon, Kuenzi and Blum2003; Wilkens et al., Reference Wilkens, Oberheide, Schröder, Azem, Steinberg and Breves2012a; Domino et al., Reference Domino, Korzec and McArt2017) – a better understanding of the exact mechanisms is urgently needed.
Sites and mechanisms of gastrointestinal calcium absorption
Paracellular calcium absorption
Gastrointestinal Ca absorption can occur via the transcellular as well as paracellular pathways (Hoenderop et al., Reference Hoenderop, Nilius and Bindels2005). Passive, paracellular absorption can take place when the chemical gradient is high enough (>6 mM on the luminal side) to overcome the electrical gradient and the barrier formed by tight junction proteins, both of which hinder the transport of cations (Bronner, Reference Bronner1987). As paracellular Ca transport is dependent on its luminal concentration, it is dominant when Ca intake is high (Bronner and Pansu, Reference Bronner and Pansu1999) – for example, when Ca is provided as a bolus or via drenching. In addition, paracellular absorption can be driven by the so-called solvent drag effect. When water is absorbed due to hydrostatic and osmotic pressure, mineral ions solubilised by water dipole–ion interactions can also pass through the paracellular pathway (Goff, Reference Goff2018). The osmotic pressure contributing, to a large extent, to the solvent drag effect depends mainly on the transepithelial Na gradient generated by Na+-K+-ATPase (Karbach, Reference Karbach1992).
Paracellular Ca transport in both directions can be found throughout the entire intestine, depending on the gradient. It is likely that the rumen multilayer epithelium is too dense to allow significant amounts of Ca to be absorbed via the interstitial fluid unless the luminal concentration of Ca is increased dramatically by additional supply. This hypothesis is also supported by the comparison of rumen Ca flux rates and mannitol flux rates that are used to estimate transepithelial movement of water (Figure 1) (Wilkens et al., Reference Wilkens, Mrochen, Breves and Schröder2011 and Reference Wilkens, Richter, Fraser, Liesegang, Breves and Schröder2012b).

Figure 1 Unidirectional flux rates (J) from mucosal to serosal (ms) and from serosal to mucosal (sm) of Ca as a function of those of mannitol (Man) in the rumen tissues of sheep (n = 20) and goats (n = 20) determined using the Ussing chamber in the absence of any electrochemical gradient. As mannitol is used as a marker for paracellular transport of water, the lack of any relationship between Ca J ms and Man J ms indicates transcellular Ca absorption. Modified from Wilkens et al. (Reference Wilkens, Mrochen, Breves and Schröder2011) and (Reference Wilkens, Richter, Fraser, Liesegang, Breves and Schröder2012b).
Although it has been demonstrated that 1,25-(OH)2D3 has an effect on the expression of several tight junction proteins (Chirayath et al., Reference Chirayath, Gajdzik, Hulla, Graf, Cross and Peterlik1998; Kutuzova and DeLuca, Reference Kutuzova and DeLuca2004), it is not clear to what extend it regulates paracellular Ca absorption. A stimulation of the expression of claudin-2 and claudin-12, tight junction proteins that increase the permeability for Ca, was found in response to long-term dietary Ca restriction in the small intestine of goats (Elfers et al., Reference Elfers, Marr, Wilkens, Breves, Langeheine, Brehm and Muscher-Banse2016b); and in CaCo-2 cells treated with 1,25-(OH)2D3, paracellular permeability was increased (Chirayath et al., Reference Chirayath, Gajdzik, Hulla, Graf, Cross and Peterlik1998).
Transcellular, pre-intestinal calcium absorption
In monogastric animals, transcellular Ca absorption mainly occurs in the duodenum and upper jejunum (Hoenderop et al., Reference Hoenderop, Nilius and Bindels2005). The cellular mechanism consists of at least three steps: Ca enters the cell via the transient receptor potential vanilloid channel type 6 (TRPV6), is bound to the cytosolic protein calbindin-D9K (CaBPD9K), translocated to the basolateral membrane and extruded mainly by the plasma membrane Ca2+-ATPase isoform 1b (PMCA1b) (Figure 2). A significant stimulation of expression by 1,25-(OH)2D3 has been shown in all three of the abovementioned structures (Hoenderop et al., Reference Hoenderop, Nilius and Bindels2005).

Figure 2 Ca transport mechanisms and transepithelial potential difference in the rumen, small intestine, the thick ascending limp of the loop of Henle (TAL), and the distal and connecting tubules (DT, CT) of the kidneys in ruminant species. PMCA, plasma membrane Ca2+-ATPase isoform 1b; TRPV6/5, transient receptor potential vanilloid channel type 6/5; CaBPD9K/CaBPD28K, calbindin-D9K/D9K; NKCC2, Na+-K+-Cl– co-transporter type 2; ROMK1, renal outer medullary K+ channel type 1; NCX, Na+/Ca2+ exchanger type 1. Explanations of the mechanisms are given in the corresponding text.
Marked differences to monogastric species have been described for ruminants, concerning the localisation and vitamin D sensitivity of Ca absorption along the gastrointestinal axis, particularly with respect to the forestomach compartment. While active Ca transport (3.0 ± 1.9 nmol/cm2·h) determined in Ussing chambers in the omasum has only been investigated and demonstrated in sheep (Höller et al., Reference Höller, Breves and Dubberke1988b), greater Ca net flux rates in the rumen were reported for sheep, goats (Figure 3) and cattle (13.8 ± 1.8 nmol/cm2·h). But as TRPV6 and CaBPD9K are not expressed in ovine, caprine and bovine rumen epithelia, this pre-intestinal Ca absorption is probably not mediated by the classical mechanism described for the intestine of monogastric animals (Höller et al., Reference Höller, Breves, Kocabatmaz and Gerdes1988a; Schröder et al., Reference Schröder, Goebel, Huber and Breves2001, Reference Schröder, Wilkens, Ricken, Leonhard-Marek, Fraser and Breves2015; Wilkens et al., Reference Wilkens, Mrochen, Breves and Schröder2011, Reference Wilkens, Richter, Fraser, Liesegang, Breves and Schröder2012bReference Schröder, Wilkens, Ricken, Leonhard-Marek, Fraser and Breves). Neither long-term dietary Ca restriction of sheep and goats nor administration of supraphysiological amounts of 1,25-(OH)2D3 resulted in increased Ca net flux rates across rumen epithelia measured in Ussing chambers (Figure 3) (Wilkens et al., Reference Wilkens, Mrochen, Breves and Schröder2011 and Reference Wilkens, Richter, Fraser, Liesegang, Breves and Schröder2012b). Hyde and Fraser estimated Ca transport in vivo by an administration of stable strontium. In contrast to the abovementioned studies, they observed that rumen Ca transport doubled after treatment of sheep with 1α-OHD3 (Hyde and Fraser, Reference Hyde and Fraser2014). However, no satisfying explanation for this inconsistency was found. It might be speculated that alterations regarding passage rate and rumen motility as a response to the hypercalcaemic effect of the treatment contribute to overall Ca transport in vivo (Daniel, Reference Daniel1983). In vitro, rumen Ca net flux rates of sheep determined in Ussing chambers seem to depend on the presence of short-chain fatty acids (SCFA; 0, 40 and 100 mmol/l in the mucosal buffer: 2.41 ± 0.55, 9.59 ± 1.55 and 19.41 ± 3.37 nmol/cm2·h) and are increased by feeding 15 g of concentrate per kilogram body weight for 3 weeks in comparison to a ration consisting of hay only (5.63 ± 0.54, 17.43 ± 0.70 and 34.54 ± 2.67 nmol/cm2·h) (Uppal et al., Reference Uppal, Wolf and Martens2003a). Therefore, an apical transport mechanism based on a Ca2+/H+ exchange system was discussed (Lutz and Scharrer, Reference Lutz and Scharrer1991; Schröder et al., Reference Schröder, Wilkens, Ricken, Leonhard-Marek, Fraser and Breves2015). Whether a higher Ca intake in the concentrate fed sheep might have altered rumen Ca transport mechanisms directly cannot be clarified. On the one hand, low luminal Ca concentrations before sacrifice did not influence the flux rates in sheep and goats (Figure 3). On the other hand, a greater contribution of pre-intestinal Ca to overall absorption was reported in a meta-analysis (Schröder and Breves, Reference Schröder and Breves2006).

Figure 3 Rumen Ca net flux rates (Jnet) of female sheep and goats aged 6 to 7 months kept on adequate (con, 0.92% and 1.10%, n = 5) or restricted Ca supply (Ca–, 0.26% and 0.22%, n = 5) treated with a placebo or fed the same diets and treated with 1,25-dihydroxyvitamin D3 (1,25-(OH)2D3, n = 5) (0.5 µg/kg body weight) 12 h before sacrifice determined in Ussing chambers in the absence of any electrochemical gradient. Means ± SEM. Modified from Wilkens et al. (Reference Wilkens, Mrochen, Breves and Schröder2011) and (Reference Wilkens, Richter, Fraser, Liesegang, Breves and Schröder2012b).
As Na transport is also – although to a lesser extent – increased by higher luminal concentrations of SCFA (Uppal et al., Reference Uppal, Wolf, Khahra and Martens2003b), rumen Ca transport could be mediated by a more complex ion exchanging mechanism. Another candidate for the apical uptake of Ca could be transient receptor potential vanilloid channel type 3 (TRPV3). In patch clamp measurements, agonists of this channel were shown to stimulate currents mediated by Ca2, NH4 and Na into HEK-293 cells expressing bovine TRPV3 (Schrapers et al., Reference Schrapers, Sponder, Liebe, Liebe and Stumpff2018). An involvement of Na transport might also explain the finding that feeding a ration negative in dietary cation–anion difference (DCAD) to sheep affects the ratio of the electroneutral to the electrogenic component of rumen Ca transport from the mucosal to the serosal side (Figure 4) (Wilkens et al., Reference Wilkens, Praechter, Breves and Schröder2016). In vivo and in vitro studies have reported both a stimulating effect of essential oils, substances that are known to interfere with TRP channels, and also interactions between the absorption of Ca, NH4 and Na. A conductance for NH4 was blocked by divalent cations in bovine rumen epithelial cells. Addition of 10 µM menthol enhanced Ca net flux rates determined for ovine rumen epithelia in Ussing chambers from 8.60 ± 1.43 to 13.24 ± 0.91 nmol/cm2·h and Na-mediated short-circuit currents (Rosendahl et al., Reference Rosendahl, Braun, Schrapers, Martens and Stumpff2016). In dairy cows, oral administration of 1.2 g essential oils with menthol as the major compound increased plasma Ca from 2.46 to 2.53 mM and decreased plasma urea from 4.28 to 3.92 mM (Braun et al., Reference Braun, Schrapers, Mahlkow-Nerge, Stumpff and Rosendahl2019).

Figure 4 Correlation between electrical driving force and unidirectional Ca fluxes (J) from mucosal to serosal (ms) and from serosal to mucosal (sm) of castrated male sheep aged 8 months kept either on a ration positive in dietary cation–anion difference (DCAD) (control, n = 4) or negative in DCAD (low DCAD, n = 5). The electroneutral component of Jms represented by the intercept of the linear function revealed by regression analysis is greater (P < 0.01) in sheep kept on a diet low in DCAD (control: Jms = 7.76 (±1.23) + 7.53 (±0.77)·ξ –0.5; low DCAD: Jms = 13.32 (±4.42) + 9.95 (±2.77)·ξ –0.5). Means ± SEM. Modified from Wilkens et al. (Reference Wilkens, Praechter, Breves and Schröder2016).
As also shown in Table 1, these findings show that rumen Ca transport depends on luminal abundance of different factors and nutrients. Therefore, greater Ca flux rates determined for rumen tissue of lactating goats in comparison to dried-off animals (2.28 ± 0.35 v. 6.75 ± 1.16 nmol/cm2·h) could be either a direct effect of lactation or be caused by the different feeding regime and/or an enlargement of the luminal surface (Starke et al., Reference Starke, Reimers, Muscher-Banse, Schröder, Breves and Wilkens2016). In cows, rumen Ca transport estimated by the administration of stable strontium is stimulated by lactation and decreased when forestomach motility is reduced (Hyde et al., Reference Hyde, Wilkens and Fraser2019). As impaired motility was observed with decreased plasma Ca concentrations (Daniel, Reference Daniel1983), inefficient ruminal Ca absorption following a disturbance of Ca mobilisation from the skeleton might aggravate hypocalcaemia in peripartum cows.
Transcellular, intestinal calcium absorption
Studies provide conflicting results on the intestinal absorption of Ca. 1,25-(OH)2D3-regulated proteins, essential for transcellular Ca absorption, have been identified in the small intestine of cattle (Yamagishi et al., Reference Yamagishi, Miyazaki and Naito2006; Schröder et al., Reference Schröder, Wilkens, Ricken, Leonhard-Marek, Fraser and Breves2015), sheep (Schröder et al., Reference Schröder, Goebel, Huber and Breves2001; Wilkens et al., Reference Wilkens, Kunert-Keil, Brinkmeier and Schröder2009, Reference Wilkens, Mrochen, Breves and Schröder2011) and goats (Wilkens et al., Reference Wilkens, Richter, Fraser, Liesegang, Breves and Schröder2012b; Elfers et al., Reference Elfers, Wilkens, Breves and Muscher-Banse2015). However, Ca transport across ovine and caprine epithelia when determined in vitro in the absence of an electrochemical gradient appears to be very low compared to monogastric animals such as horses using the same methods (Figure 5) (Wilkens et al., Reference Wilkens, Marholt, Eigendorf, Muscher-Banse, Feige, Schröder, Breves and Cehak2017). In the colon, Ca net flux rates are also very low. As in the rumen, significant active Ca transport (6.55 ± 2.01 nmol/cm2·h) across the colon of sheep is only detectable in the presence of SCFA. Unfortunately, no published data are available on intestinal Ca transport determined for bovine epithelia.
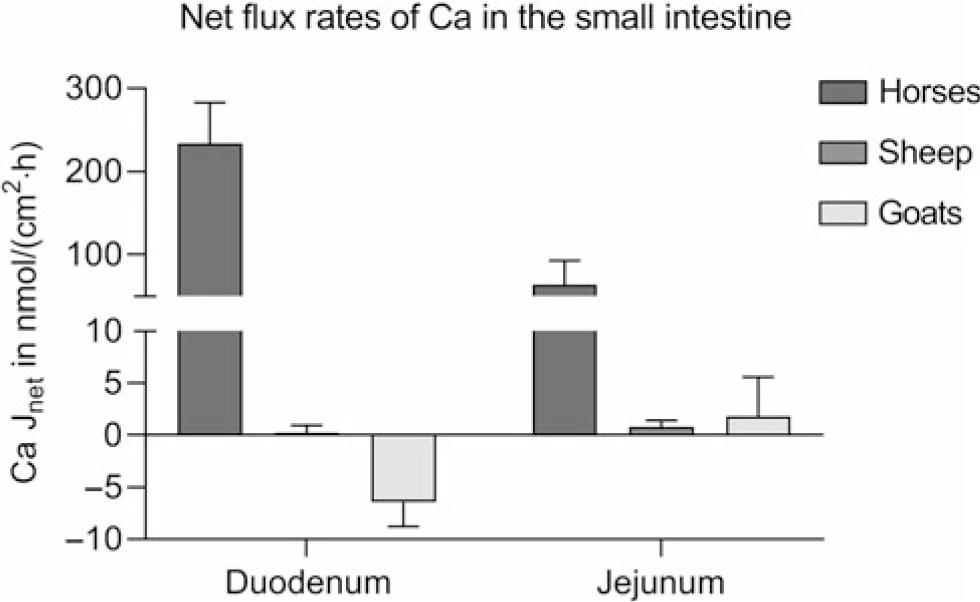
Figure 5 Intestinal Ca net flux rates (Jnet) of horses of both sexes, aged 3 to 22 years (n = 10), female sheep (n = 5) and female goats (n = 5) aged 6 to 7 months kept on adequate Ca supply determined in Ussing chambers in the absence of any electrochemical gradient. Means ± SEM. Modified from Wilkens et al. (Reference Wilkens, Mrochen, Breves and Schröder2011), (Reference Wilkens, Richter, Fraser, Liesegang, Breves and Schröder2012b) and (Reference Wilkens, Marholt, Eigendorf, Muscher-Banse, Feige, Schröder, Breves and Cehak2017).
In goats kept on a low Ca diet or treated with vitamin D, duodenal Ca flux rates measured in Ussing chambers were significantly increased in some (Wilkens et al., Reference Wilkens, Richter, Fraser, Liesegang, Breves and Schröder2012b) but not in all studies (Schröder et al., Reference Schröder, Rittmann, Pfeffer and Breves1997; Sidler-Lauff et al., Reference Sidler-Lauff, Boos, Kraenzlin and Liesegang2010). Higher flux rates and a more pronounced stimulation of transcellular Ca transport by dietary Ca restriction was accompanied by an increase in RNA, and protein expression of TRPV6 could be shown for the jejunum of goats indicating that this segment is more active for overall Ca absorption (Figure 6a) (Wilkens et al., Reference Wilkens, Richter, Fraser, Liesegang, Breves and Schröder2012b; Elfers et al., Reference Elfers, Wilkens, Breves and Muscher-Banse2015). Although the efficiency of net Ca absorption from the jejunum, measured by applying the Thiry-Vella loop technique, was increased in sheep with dietary Ca restriction (Abdel-Hafeez et al., Reference Abdel-Hafeez, Manas-Almendros, Ross, Care and Marshall1982), this could not be demonstrated in protein expression studies and Ussing chamber experiments (Figure 6b) (Wilkens et al., Reference Wilkens, Mrochen, Breves and Schröder2011). In goats kept on a reduced protein diet, the intestinal absorption of Ca was diminished with a concomitant reduction of CaBPD9K and PMCA1b, probably caused by decreased 1,25-(OH)2D3 concentrations (Figure 6c) (Elfers et al., Reference Elfers, Wilkens, Breves and Muscher-Banse2015).

Figure 6 Intestinal Ca net flux rates (Jnet) of female sheep and goats aged 6 to 7 months kept on adequate (control, 0.92% and 1.10%, n = 5) or restricted Ca supply (Ca restriction, 0.26% and 0.22%, n = 5) and male goats aged 3 to 4 months kept on adequate (control, 22% CP, n = 7) or restricted n supply (n restriction, 8% CP, n = 6) determined in Ussing chambers in the absence of any electrochemical gradient. Significant differences revealed by the Student’s t test are marked with asterisks. Means ± SEM; *, P < 0.05. Modified from Elfers et al. (Reference Elfers, Wilkens, Breves and Muscher-Banse2015), Wilkens et al. (Reference Wilkens, Mrochen, Breves and Schröder2011) and (Reference Wilkens, Richter, Fraser, Liesegang, Breves and Schröder2012b).
Taken together with results from lactating and dried-off sheep and goats, it might be concluded that the responsiveness of intestinal Ca absorption to enhanced demand or restricted supply varies between different species and ages (Wilkens et al., Reference Wilkens, Breves and Schröder2014; Klinger et al., Reference Klinger, Schröder, Gemmer, Reimers, Breves, Herrmann and Wilkens2016; Starke et al., Reference Starke, Reimers, Muscher-Banse, Schröder, Breves and Wilkens2016). In lactating and non-lactating cows, balance studies demonstrated that Ca digestibility is not increased with dietary Ca restriction, although lactation itself seems to enhance gastrointestinal absorption (Table 1). However, a full adaptation to increased Ca demand during lactation seems to take at least 2 days (van´t Klooster, Reference van´t Klooster1976).
Salivary secretion of phosphorus
As rumen Pi concentrations play a pivotal role for rumen buffering, fermentation and microbial protein synthesis, large amounts of Pi are secreted with saliva and resorbed in the lower digestive tract. Rumen Pi concentration thus depends on dietary P intake and the rate of salivary Pi secretion (Breves and Schröder, Reference Breves and Schröder1991). The role of salivary Pi is also reflected by the observation that salivary Pi concentrations and expression of NaPi IIb (SLC34A2) and Pi transporter PiT1 (SLC20A1), both Na-dependent Pi transporters, increase with age, that is, with the development of the gastrointestinal tract (Huber et al., Reference Huber, Roesler, Muscher, Hansen, Widiyono, Pfeffer and Breves2003). Interestingly, significant differences in both rumen Pi concentrations (see below) and salivary Pi were found when adult sheep (11.3 ± 1.2 mM) and goats (23.1 ± 3.2 mM) were kept on the same ration, indicating species differences in respect to salivary Pi secretion (Wilkens et al., Reference Wilkens, Breves and Schröder2014).
Data on the regulation of salivary Pi secretion are inconsistent. Furthermore, data on potential molecular regulatory mechanisms of Pi transport in salivary glands are lacking. Intravenous loading with Pi resulted in an increase in Pi secretion via the parotid gland of sheep and cows, indicating plasma Pi concentration is the most important factor (Scott and Beastall, Reference Scott and Beastall1978; Riad et al., Reference Riad, Lefaivre and Barlet1987). In goats and sheep, the administration of PTH induced an increase in saliva Pi concentration in some studies (Wright et al., Reference Wright, Blair-West, Nelson and Tregear1982; Isac et al., Reference Isac, Valverde, Lupiani, Martinez de Victoria and Manas1989), while others found a decreasing effect (Mañas-Almendros et al., Reference Mañas-Almendros, Ross and Care1982). An injection of exogenous 1,25-(OH)2D3 reduced salivary Pi concentrations in sheep and cows (Mañas-Almendros et al., Reference Mañas-Almendros, Ross and Care1982; Riad et al., Reference Riad, Lefaivre and Barlet1987). A possible explanation for these contradictions could be the alteration of saliva flow rate, which is difficult to be addressed in the experimental design (Isac et al., Reference Isac, Valverde, Lupiani, Martinez de Victoria and Manas1989). The salivary flow rate is mainly regulated by the physical nature of the diet fed (Wilson and Tribe, Reference Wilson and Tribe1963). Pelleted diets induced lower daily saliva flow rates than chopped or long hay based on less chewing (Duric et al., Reference Duric, Zhao, Orskov and Chen1994).
However, rumen Pi concentrations were significantly increased in sheep (24.2 ± 1.0 v. 28.0 ± 0.9 mM) and goats (42.1 ± 2.9 v. 50.0 ± 3.8 mM) kept on a Ca-restricted ration for several weeks that led to an increase in an endogenous production of 1,25-(OH)2D3, even though plasma (1.97 ± 0.13 v. 1.87 ± 0.21 mM) and salivary concentrations of Pi (37.3 ± 3.4 v. 37.0 ± 1.2 mM) were not affected by this feeding regime in goats (Wilkens et al., Reference Wilkens, Richter, Fraser, Liesegang, Breves and Schröder2012b, Reference Wilkens, Breves and Schröder2014).
Sites and mechanisms of gastrointestinal phosphorus absorption
The absorption of Pi takes place along the whole of the intestinal tract. In principle, Pi absorption can be divided into a passive paracellular process and a saturable, active transcellular process. In vivo studies with the temporarily isolated reticulo-rumen from sheep demonstrated a positive linear relationship between rumen Pi concentrations and net Pi disappearance, indicating passive paracellular absorption of Pi. No indications of active Pi transport or saturation phenomena could be determined (Breves et al., Reference Breves, Höller, Packheiser, Gabel and Martens1988; Beardsworth et al., Reference Beardsworth, Beardsworth and Care1989). In vitro studies with rumen epithelium confirmed that no Pi net flux was found under short-circuit conditions, that is, in the absence of any electrochemical gradient, in Ussing chamber experiments (Breves et al., Reference Breves, Höller, Packheiser, Gabel and Martens1988). A passive process of Pi absorption also occurs in the omasal epithelium of sheep (Höller et al., Reference Höller, Breves and Dubberke1988b). However, balance studies clearly indicate that there is no net absorption from but a substantial secretion of Pi into the forestomach in vivo (Table 1).
In ruminants as in monogastric species, the small intestine is the major site for Pi absorption (Pfeffer et al., Reference Pfeffer, Thompson and Armstrong1970). Dietary P concentration and 1,25-(OH)2D3 are the main regulators of intestinal Pi transport in monogastric species. Paracellular Pi transport across the intercellular spaces of the small intestines has been postulated. However, no potential candidate genes which might mediate such mechanisms have been identified.
An H+-dependent Pi co-transport into duodenal brush border membrane vesicles (BBMV) from sheep and cattle was demonstrated, and this was stimulated by low dietary P (Shirazi-Beechey et al., Reference Shirazi-Beechey, Kemp, Dyer and Beechey1989, Reference Shirazi-Beechey, Beechey, Penny, Vayro, Buchan and Scott1991). In the jejunum of sheep, the saturation of Pi absorption was demonstrated with the use of a Thiry-Vella loop when the infused solution was as high as 15 mM of Pi (Care et al., Reference Care, Barlet and Abdel-Hafeez1980). Interestingly, studies on jejunal unidirectional Pi flux rates in Ussing chambers using intestinal tissue from sheep and goats demonstrated a substantial part of active Pi transport which was inhibited by arsenate by a reduction of luminal Na+ concentrations and by serosal addition of ouabain. This Na+-dependent Pi co-transport could be stimulated by dietary P depletion, while changes in vitamin D metabolism were not involved (Schröder et al., Reference Schröder, Kappner, Failing, Pfeffer and Breves1995). This provides evidence for an active Pi transport mechanism like that in non-ruminant species, with the highest absorption rates being found in the ileum of young goats (about 3 to 4 months) and adult sheep (Schröder et al., Reference Schröder, Kappner, Failing, Pfeffer and Breves1995; Elfers et al., Reference Elfers, Wilkens, Breves and Muscher-Banse2015). To confirm that active Pi transport is Na+-dependent, Pi uptake studies into isolated BBMV from goat jejunum were performed under different conditions of extravesicular Na+ and H+ concentrations (Schröder and Breves, Reference Schröder and Breves1996). The results are similar to data from monogastric species and showed that a major proportion of jejunal Pi uptake is Na+-dependent, and can be stimulated by H+, in contrast to duodenal Pi transport which is H+-dependent and Na-sensitive.
After the molecular identification of an intestinal Na+-dependent Pi transporter in mice (NaPi IIb) (Hilfiker et al., Reference Hilfiker, Hattenhauer, Traebert, Forster, Murer and Biber1998), it could be shown that caprine NaPi IIb expression corresponded to murine NaPi IIb (Huber et al., Reference Huber, Walter, Schröder, Biber, Murer and Breves2000). Both NaPi IIb mRNA and protein were absent in the duodenum of goats, while NaPi IIb was strongly expressed in the jejunum (Huber et al., Reference Huber, Walter, Schröder and Breves2002). With jejunal BBMV, it could be shown that a high linear correlation exists between transport capacity for Pi and NaPi IIb protein expression, indicating that the majority of Na-dependent Pi transport was mediated by NaPi IIb. Furthermore, the existence of an additional electrogenic Na-dependent Pi transporter, called PiT1, was shown in the small intestine of goats (Figure 7) (Elfers et al., Reference Elfers, Wilkens, Breves and Muscher-Banse2015). PiT1 belongs to the Pi transporter family that uses either Na or H+ gradients to transport Pi (Saier, Reference Saier2000). The mechanism for extrusion of Pi is still under investigation. In Holstein cows, the highest NaPi IIb RNA expression was found in the distal jejunum and ileum, while the expression in the upper intestinal segments was nearly absent (Foote et al., Reference Foote, Lambert, Brady and Muir2011).

Figure 7 Pi transport mechanisms and transepithelial potential difference in the small intestine and proximal tubule of the kidneys in ruminant species. Apical entry occurs through Na-dependent Pi transporter family (NaPi) subtypes IIa and IIc or IIb and Na-dependent phosphate transporter 1 (PiT1). Basolateral extrusion mechanism of Pi is currently unknown. Further explanations of the mechanisms are given in the corresponding text.
To characterise Pi transport in the duodenum in more detail, transepithelial Pi flux rates have been performed in Ussing chamber experiments, in the presence or absence of mucosal Na at different pH levels. From these studies, it could be concluded that at least two different Pi transport mechanisms exist in the goat intestinal tract which are regionally separate: in the duodenum, Pi uptake is mainly mediated by an H+-dependent Na-sensitive mechanism, while in the jejunum, Pi uptake is mainly Na-dependent and H+-sensitive (Schröder et al., Reference Schröder, Kappner, Failing, Pfeffer and Breves1995; Huber et al., Reference Huber, Walter, Schröder and Breves2002).
In lactating goats, Pi flux rates from the mucosal to the serosal side of the epithelium, determined in the duodenum and jejunum, were significantly smaller in comparison to dried-off animals, resulting in a reduced net absorption (Figure 8). This was accompanied by a downregulation of jejunal NaPi IIb, both on RNA and protein levels, probably as a consequence of either higher P intake or enhanced mobilisation of Pi from the skeleton (Starke et al., Reference Starke, Reimers, Muscher-Banse, Schröder, Breves and Wilkens2016). In line with other studies, NaPi IIb was not detectable in the duodenum (Huber et al., Reference Huber, Walter, Schröder and Breves2002). An ontogenetic study with goats found that Pi-binding properties changed during the development of the gastrointestinal tract of growing animals, indicating that alterations of NaPi IIb and/or PiT1 must be taking place (Huber et al., Reference Huber, Roesler, Muscher, Hansen, Widiyono, Pfeffer and Breves2003).

Figure 8 Unidirectional, duodenal (Duo) and jejunal (Jeju) Pi flux rates (J) from serosal to mucosal (sm) and from mucosal to serosal (ms) of dried-off (n = 6) and lactating goats (n = 6) determined in Ussing chambers in the absence of any electrochemical gradient. Significant differences revealed by the Student’s t test are marked with asterisks. Means ± SEM; *, P < 0.05. Modified from Starke et al. (Reference Starke, Reimers, Muscher-Banse, Schröder, Breves and Wilkens2016).
Interestingly, dietary P depletion modifies the intestinal absorption of Pi in young goats (4 to 5 months) but without the involvement of vitamin D metabolism (Schröder et al., Reference Schröder, Kappner, Failing, Pfeffer and Breves1995). Therefore, an unknown Pi-sensing mechanism is hypothesised in the small intestine of ruminant species. Even when 1,25-(OH)2D3 concentrations were altered during dietary protein reduction, modulation of the expression of NaPi IIb and PiT1 in the small intestine was not found (Elfers et al., Reference Elfers, Wilkens, Breves and Muscher-Banse2015). These results contrast with data from monogastric species where a 1,25-(OH)2D3-dependent regulation of NaPi IIb was found (Murer et al., Reference Murer, Forster and Biber2004).
In young lambs (1 week old), the efficacy of Pi absorption from the colon was almost the same as in the upper and mid-jejunum, but the velocity of Pi absorption decreased during subsequent development (Scharrer, Reference Scharrer1985). In adult sheep fitted with re-entrant cannulae, the proximal colon was perfused with an electrolyte solution free of Pi, and net Pi secretion was determined. Net absorption of Pi from the colon was shown when Pi concentrations of the electrolyte solution were between 2.5 and 6.5 mM (Höller et al., Reference Höller, Figge, Richter and Breves1988c).
Renal handling of calcium
In the kidneys of rats and hamsters, 70% of filtered Ca is resorbed paracellulary in the proximal tubules, while up to 20% is resorbed in the thick ascending limb of the loop of Henle (TAL) (Lassiter et al., Reference Lassiter, Gottschalk and Mylle1963). In the proximal tubules, where an osmotic gradient is built up due to the resorption of Na, glucose and amino acids, paracellular Ca transport is driven mainly by the solvent drag effect (Friedman and Gesek, Reference Friedman and Gesek1995). In TAL, a lumen-positive transepithelial potential difference is generated by the electroneutral uptake of Na, K and Cl via the Na+-K+-2Cl–-co-transporter (NKCC) followed by the basolateral extrusion of Cl and the apical secretion of K. Tight junctions in this segment contain claudin-16 that increases cation permeability, claudin-19 that blocks anion permeability, and claudin-14 that decreases cation permeability mediated by claudin-16 (Negri, Reference Negri2015). Interestingly, we observed a downregulation of claudin-19 with dietary Ca restriction in sheep and goats, which contrasts findings in rats (Frick et al., Reference Frick, Asplin, Favus, Culbertson, Krieger and Bushinsky2013), and an upregulation of claudin-16 during lactation in goats (unpublished results).
Active, transcellular, 1,25-(OH)2D3-regulated Ca transport is found in the distal and connecting tubules. For active resorption of Ca, a transport mechanism similar to that generally accepted for the small intestine (TRPV5, calbindin-D28K and basolateral extrusion by the Na+/Ca2+ exchanger NCX1) has been described (Figure 2) (Hoenderop et al., Reference Hoenderop, Dardenne, van Abel, van der Kemp, van Os, St. Arnaud and Bindels2002). In rodents fed a diet low in Ca, there was an increase in RNA expression of TRPV5 and CaBPD28K (Hoenderop et al., Reference Hoenderop, Dardenne, van Abel, van der Kemp, van Os, St. Arnaud and Bindels2002; Ko et al., Reference Ko, Lee, Vo, Jung, Choi, Cheung, Kim, Park, Oh and Jeung2009). Furthermore, it was demonstrated in mice that lactation stimulated renal RNA expression of TRPV5 and CaBPD28K (van Cromphaut et al., Reference van Cromphaut, Rummens, Stockmans, van Herck, Dijcks, Ederveen, Carmeliet, Bouillon and Carmeliet2003). For adult sheep and goats, we found that ruminant kidney does not respond to a challenge of Ca homeostasis by altered expression of structures mediating Ca resorption. With respect to CaBPD28K, we even observed a downregulation in dietary Ca-restricted or lactating goats, instead of the stimulation that has been reported for mice (Herm et al., Reference Herm, Muscher-Banse, Breves, Schröder and Wilkens2015). Interestingly, in lactating goats, urinary Ca excretion was not increased. We speculated that enhanced resorption in TAL mediated by prolactin and/or PTHrP might have compensated for the downregulation of TRPV5, CaBPD28K and NCX1 RNA expressions in the distal parts of nephron (Herm et al., Reference Herm, Muscher-Banse, Breves, Schröder and Wilkens2015). Our findings on the structural level regarding animals kept on a low Ca diet could be explained by characteristically low renal Ca excretion in adult ruminants that cannot be further diminished when Ca homeostasis is challenged. As in cattle and lactating cows (Table 1), fractional excretion of Ca was not reduced by dietary Ca restriction in small ruminants (sheep: 0.83 ± 0.22 v. 1.06 ± 0.24%, goats: 0.71 ± 0.13 v. 1.03 ± 0.21%).
However, in young goats (3 to 4 months) kept on a Ca-reduced diet, a stimulation of CaBPD28K and NCX1 RNA expression occurred based on elevated 1,25-(OH)2D3 levels. This is in line with data from balance studies conducted with goat kids (Table 1). A concomitant decrease in dietary Ca and protein in these young animals caused a decrease in 1,25-(OH)2D3 concentrations, resulting in a downregulation of TRPV5, CaBPD28K and NCX1 protein expressions (Firmenich et al., Reference Firmenich, Elfers, Wilkens, Breves and Muscher-Banse2018).
A way to increase renal Ca excretion in ruminants is to feed a ration negative in DCAD (Table 1). This feeding regime induces a compensated acidosis that results in increased tissue responsiveness to PTH (Goff et al., Reference Goff, Liesegang and Horst2014). In addition, DCAD treatment leads to significant changes in Ca balance before parturition. Several studies in dairy cows have demonstrated that urinary pH is decreased, while renal excretion of Ca is increased up to 10-fold (0.4 ± 0.2 v. 4.1 ± 0.9 g per day) (Grünberg et al., Reference Grünberg, Donkin and Constable2011; Wilkens et al., Reference Wilkens, Oberheide, Schröder, Azem, Steinberg and Breves2012a). As TRPV5 activity is pH-dependent, increased renal Ca excretion might be caused by a direct inhibitory effect of tubular acidosis on renal resorption of Ca as shown in the kidney of dogs and for rabbit TRPV5 (Sutton et al., Reference Sutton, Wong and Dirks1979; Yeh et al., Reference Yeh, Sun, Lee, Chen and Huang2003). In preliminary experiments conducted with sheep, we observed that the expression of TRPV5, CaBPD28K and NCX1 was not significantly altered under these conditions (unpublished results). Assuming that this occurs in cows kept on a low DCAD ration, too, this might indicate that renal resorption is immediately restored when the ration is changed postpartum. An adaptation on the functional level occurs faster than the stimulation of gene expression and could contribute to the beneficial effects of a low DCAD diet in peripartum cow.
Renal handling of phosphorus
During normophosphataemia, about 98% to 99% of filtered Pi is resorbed in the kidneys of ruminant species (Widiyono et al., Reference Widiyono, Huber, Failing and Breves1998). The mean plasma threshold for renal Pi excretion in goats lies around 4.3 mM (Widiyono et al., Reference Widiyono, Huber, Failing and Breves1998). Filtered Pi is reabsorbed mainly by the proximal tubule cells. The uptake of filtered Pi at the apical side is mediated by Na+-dependent Pi transporters: electrogenic NaPi IIa (SLC34A1) and electroneutral NaPi IIc (SLC34A3) in both ruminant and non-ruminant species (Figure 7) (Biber and Murer, Reference Biber and Murer1994; Shirazi-Beechey et al., Reference Shirazi-Beechey, Penny, Dyer, Wood, Tarpey, Scott and Buchan1996; Huber et al., Reference Huber, Roesler, Muscher, Hansen, Widiyono, Pfeffer and Breves2003; Starke et al., Reference Starke, Cox, Sudekum and Huber2013). Ovine and caprine amino acid sequence, kinetic and stoichiometric parameters of renal cortex Na+-dependent Pi transport are comparable to the type IIa Na+/Pi co-transport in monogastric species (Schröder et al., Reference Schröder, Walter, Breves and Huber2000). Basolateral Pi extrusion mechanism is still unknown.
Changes in dietary P intake and consequent changes in extracellular Pi and PTH are the main regulators of renal Pi transporters in monogastric species (Biber et al., Reference Biber, Murer and Forster1998). In mature goats and sheep on a P-reduced diet, no changes in renal transport capacities (Schröder et al., Reference Schröder, Walter, Breves and Huber2000) or on NaPi IIa expression (Huber et al., Reference Huber, Roesler, Holthausen, Pfeffer and Breves2007) were determined. In contrast, dietary P restriction altered urinary Pi excretion in goat kids (Table 1). In young goats (3 to 4 months) on a high P diet, there was a decrease in renal Pi reabsorption capacity and an internalisation of NaPi IIa occurred (Huber et al., Reference Huber, Roesler, Holthausen, Pfeffer and Breves2007; Muscher et al., Reference Muscher, Hattendorf, Pfeffer, Breves and Huber2008). Strong correlations between NaPi IIa mRNA and plasma Pi as well as plasma PTH concentrations indicated that elevated Pi and high PTH concentrations were able to modulate renal Pi excretion by reducing Pi reabsorption (Muscher et al., Reference Muscher, Hattendorf, Pfeffer, Breves and Huber2008). This phenomenon is different to that in monogastric animals where NaPi IIa expression was decreased only at the protein level (Murer et al., Reference Murer, Forster, Hernando, Lambert, Traebert and Biber1999).
Besides dietary P and PTH, a reduction in dietary protein also modulates mineral homeostasis in young goats (4 to 5 months) (Muscher et al., Reference Muscher, Piechotta, Breves and Huber2011). A significant increase in NaPi IIa expression and a concomitant decrease in PTH receptor expression were observed in young goats (4 to 5 months) when dietary protein was diminished. The concentration of 1,25-(OH)2D3 was reduced while PTH levels were not affected (Starke et al., Reference Starke, Cox, Sudekum and Huber2013; Starke and Huber, Reference Starke and Huber2014; Firmenich et al., Reference Firmenich, Elfers, Wilkens, Breves and Muscher-Banse2018). The stimulation of NaPi IIa expression during a protein-reduced diet is not obvious. It was postulated that a reduction in Pi concentrations in the ultrafiltrate stimulated the expression of NaPi IIa in apical membranes. The decline in Pi in the ultrafiltrate could be caused by a drop in the glomerular filtration rate (GFR) to conserve urea because a reduction in GFR by 60% was detected in goats fed a low protein diet (Eriksson and Valtonen, Reference Eriksson and Valtonen1982; Valtonen et al., Reference Valtonen, Uusi-Rauva and Eriksson1982). Therefore, an unknown Pi-sensing mechanism(s) in the proximal tubules must exist. Interestingly, a stimulation of NaPi IIa expression was accomplished by dietary protein reduction and thereby, presumably, a reduction in Pi in the ultrafiltrate. A direct dietary Pi depletion without manipulation of GFR did not show the same effects (Schröder et al., Reference Schröder, Walter, Breves and Huber2000).
In pre-ruminant animals, the kidneys are the main excretory pathway for an excess of Pi. During the development of the rumen, changes occurred. When the threshold of plasma Pi exceeded, renal elimination of Pi is neither stimulated nor eliminated, but more Pi is secreted in the saliva to the rumen, where it is used by microorganisms. Therefore, PTH-mediated regulation of renal Pi excretion is less important in adult ruminants than in growing ruminants.
In adult ruminants, renal Pi excretion does not seem to be regulated. An intravenous infusion of PTH did not alter renal excretion of Pi in sheep, and a dietary Ca restriction for several weeks did not affect fractional excretion of Pi in small ruminants (sheep: 1.23 ± 0.23% v. 0.82 ± 0.07%, goats: 2.26 ± 0.0.80% v. 2.79 ± 0.80%) (Clark et al., Reference Clark, French, Beal, Cross and Budtz-Olsen1975; Herm et al., Reference Herm, Muscher-Banse, Breves, Schröder and Wilkens2015). This is in line with former results from sheep and cows (Braithwaite, Reference Braithwaite1975; Taylor et al., Reference Taylor, Knowlton, McGilliard, Swecker, Ferguson, Wu and Hanigan2009).
Conclusions and perspectives
The regulation of mineral homeostasis in ruminants differs not only from monogastric animals but also between and within ruminant species. Although the molecular structures that are involved in Ca and Pi transports in the intestinal tract and the kidneys have been characterised in several ruminant species, the modulation of these by different dietary interventions, by the supply of other minerals and nutrients, or as a consequence of hormonal changes in 1,25-(OH)2D3, FGF23, PTH or calcitonin are still under investigation. Ruminal Ca transport mechanisms are still not clarified. In addition, more information is required in respect to the contribution of salivary mineral secretion and bone turnover. Further research is also needed to better understand imbalances of mineral homeostasis, such as hypocalcaemia and the capacities of ruminants to adapt to marginal mineral supply when kept on P-deficient pasture. In this regard, the interplay between mineral homeostasis, availability and digestibility of nutrients and metabolic pathways regulating energy and protein metabolism should be elucidated as they are important for lactating cows as well as animals kept for meat production.
Acknowledgements
The authors wish to thank Prof. David R. Fraser for proofreading the manuscript. The presented research was partly supported by the Deutsche Forschungsgemeinschaft (Schr 342/8-1, Schr 342/8-2, Wi 3668/1-1, Mu 3585/1-1, Mu 3585/1-3) and the H. Wilhelm Schaumann Foundation.
M. R. Wilkens 0000-0003-1734-5024
A. S. Muscher-Banse 0000-0002-4149-7987
Declaration of interest
The authors declare that there are no conflicts of interest.
Ethics statement
None.
Software and data repository resources
None of the data were deposited in an official repository.