Implications
Antimicrobials are widely used worldwide in swine production to prevent the occurrence of post-weaning diarrhea. The present review explores the wide-ranging effects of tannins as a promising alternative to antimicrobials. The data presented herein could help mitigate post-weaning diarrhea problems for pig producers, including those in organic farming operations. For researchers, this review highlights potential areas for further investigation.
Introduction
Early and abrupt weaning as practiced in pig farms is a critical period of dietary transition and environmental and social upheaval for piglets, and is associated with numerous physiological, immunological and microbiological changes to the gastrointestinal tract. During this stressful period, piglets are more susceptible to infection by enteropathogens, the most widespread being enterotoxigenic Escherichia coli (ETEC). The combination of gastrointestinal disturbances occurring around weaning often leads to post-weaning diarrhea (PWD). Across Europe, ETEC is detected in 60% of PWD-affected farms (Luppi et al., Reference Luppi, Gibellini, Gin, Vangroenweghe, Vandenbroucke, Bauerfeind, Bonilauri, Labarque and Hidalgo2016). The number seems to be lower in Switzerland, with 37% of pig husbandry operations reporting problems with PWD (Hartmann, Reference Hartmann2016). The worsened productive performances, that is, the high morbidity and the depressed growth rate, as well as the increased medical costs related to PWD, result in substantial economic losses to farmers (Fairbrother et al., Reference Fairbrother, Nadeau and Gyles2005). Excluding treatment costs, Sjölund et al. (Reference Sjölund, Zoric and Wallgren2014) estimated annual costs for low-grade PWD of 40 euros per sow.
ETEC colonizes the gastrointestinal tract by binding to the apical side of enterocytes via F4 (or K88) or F18 fimbrial adhesion to receptors; once attached, the bacteria secrete the enterotoxins heat-labile toxin (LT) and heat-stable toxins a and b (STa and STb). These toxins trigger an intracellular signaling cascade resulting in the hypersecretion of electrolytes and water into the intestinal lumen, leading to watery diarrhea (Heo et al., Reference Heo, Opapeju, Pluske, Kim, Hampson and Nyachoti2013). Lekagul et al. (Reference Lekagul, Tangcharoensathien and Yeung2019) reported that the prophylactic use of in-feed antimicrobials, although strongly discouraged, remains a common practice for coping with PWD in some countries.
The extensive use of in-feed antimicrobials has been associated with an increasing incidence of antimicrobial resistance, with 70% of the pathogenic E. coli isolated from Swiss pig farms displaying resistance to at least one antimicrobial (Brand et al., Reference Brand, Gobeli and Perreten2017). Across Europe, the situation is similar. In 2017, 61% of indicator E. coli from the fecal content of fattening pigs exhibited resistance to one or more antimicrobials, with some countries in southern Europe (e.g. Spain, Italy and Portugal) having >90% of resistant isolates (European Food Safety Authority and European Centre for Disease Prevention Control, 2019). This increasing incidence of resistances gives rise to animal and public health concerns and consequently increases the political pressure to reduce antimicrobial use in pig production and generally in livestock. Although considerable efforts have been achieved in recent years to reduce antimicrobial use in Europe, solutions are still needed (European Medicines Agency, 2018). The development of alternative approaches that could contribute to optimal gut health is a prerequisite to minimize the prophylactic use of antimicrobials and could ultimately lead to the improved productivity, health and welfare of pigs, as well as ensure the long-term sustainability of pig production. The two recent decisions of the European Union (EU) to ban within the next 5 years the use of zinc oxide, added at medicinal levels in piglet feed, and the prophylactic use of antimicrobials to prevent PWD, emphasize the urgent need for alternatives. Recent, well-supported research delineates many PWD management strategies, including genetic selection for ETEC-resistant piglets devoid of F4-receptors, vaccination and nutritional approaches (Fairbrother et al., Reference Fairbrother, Nadeau and Gyles2005; Heo et al., Reference Heo, Opapeju, Pluske, Kim, Hampson and Nyachoti2013). Nutritional approaches, such as the management of protein sources and levels in the diet and the use of feed additives (prebiotics, probiotics and organic acids), seem to be the most cost-effective solutions currently used, although the effectiveness of some of these strategies remains somewhat doubtful (Lauridsen et al., Reference Lauridsen, Højberg, Kongsted and Canibe2017). For several years, a growing interest in the use of bioactive compounds from plants as alternatives to antibiotics has emerged, in concurrence with a drive toward sustainable production strategies and consumer acceptability. Bioactive compounds are secondary plant metabolites that elicit pharmacological or toxicological effects on organisms (Bernhoft, Reference Bernhoft and Bernhoft2010). Polyphenols are one of the largest groups of bioactive compounds in the plant kingdom, with more than 8000 known phenolic structures. Among polyphenols, tannins are widely studied for their antiviral, insecticidal, nematicidal, antifungal, antibacterial and antioxidant properties and might therefore represent one solution to tackle PWD (Barbehenn and Constabel, Reference Barbehenn and Constabel2011; Hoste et al., Reference Hoste, Torres-Acosta, Sandoval-Castro, Mueller-Harvey, Sotiraki, Louvandini, Thamsborg and Terrill2015; Smeriglio et al., Reference Smeriglio, Barreca, Bellocco and Trombetta2017).
This review aims to provide an overview of the large diversity of tannins and their possible contributions to the reduction of coliform PWD and improvement in and maintenance of gut health. A definition of tannin chemical structures and the wide range of their effects on bacteria, with a special emphasis on ETEC-related PWD, is explored from existing in vitro and in vivo studies. The factors affecting the bioactivity of tannins are also discussed.
Tannins, a diverse family of bioactive compounds
Definition, localization and roles in plants
Tannins are a heterogeneous group of astringent polyphenolic biomolecules that can interact with and precipitate macromolecules, such as proteins, gelatins, polysaccharides and alkaloids. Plants synthesize these compounds in mixtures that cover a wide range of molecular weights, and up to 20 000 Da have been reported (Khanbabaee and van Ree, Reference Khanbabaee and van Ree2001). Commonly, tannins are classified into three categories: condensed tannins (CTs), hydrolyzable tannins (HTs) and complex tannins.
Many tannin compounds are found in flowers, leaves, seeds, fruits, roots and tree bark samples (Sieniawska and Baj, Reference Sieniawska, Baj, Badal and Delgoda2017). The CTs appear to be stored inside tannosomes, a chloroplast-derived organelle enclosed within tonoplasts inside plant vacuoles. In these tannosomes, tannins do not interact with proteins and consequently do not interfere with plant metabolism (Brillouet et al., Reference Brillouet, Romieu, Schoefs, Solymosi, Cheynier, Fulcrand, Verdeil and Conéjéro2013). It has been shown that HTs may be synthesized and deposited in chloroplasts but also in cell walls or the intercellular space (Grundhöfer and Gross, Reference Grundhöfer and Gross2001).
The role of CTs is to defend the plant against predation by herbivore and insects primarily by reducing plant palatability, particularly in young leaves (Barbehenn and Constabel, Reference Barbehenn and Constabel2011). In roots, CTs are a chemical barrier against penetration and colonization by pathogens; in seeds, they maintain plant dormancy and have bactericidal properties (Constabel et al., Reference Constabel, Yoshida and Walker2014).
Classifications of tannins
The most widespread CTs, also known as proanthocyanidins, are oligomers (2 to 10 monomers) or polymers (>10 monomers) of flavan-3-ol units. When subjected to acidic alcohol treatment, CTs degrade to anthocyanidins, the pink-purple pigments responsible for flower coloring (hence the name). Common flavan-3-ols are [epi]catechin, [epi]afzelechin, [epi]gallocatechin, [epi]fisetinidol and [epi]robinetinidol, in accordance with the position of their –OH and –H groups, which give rise to procyanidins, prodelphinidins, profisetinidins and prorobinetinidins (Figure 1). For instance, procyanidins contain catechin or epicatechin, and prodelphinidins contain gallocatechin or epigallocatechin (Figure 1). In plants, a mixture of the aforementioned classes is often present and varies according to growing conditions (growth stage, temperature, etc.) and mode of conservation (fresh, wilted, etc.) (Girard, Reference Girard2016). The size of the CT molecule is characterized by its degree of polymerization. In addition to differences in their chemical groups, flavan-3-ols differ in their stereoisomerism: catechin and gallocatechin have a trans configuration, whereas epicatechin and epigallocatechin have a cis configuration at the C2–C3 bond. The interflavan linkages between flavan-3-ols units can be either A-type or B-type (Girard, Reference Girard2016). A-type linkages comprise C4β–C8 bonds and C2β–O–C7 or C2β–O–C5 ether bonds. B-type linkages consist of C4β–C8 or C4β–C6 linkages (Figure 1).
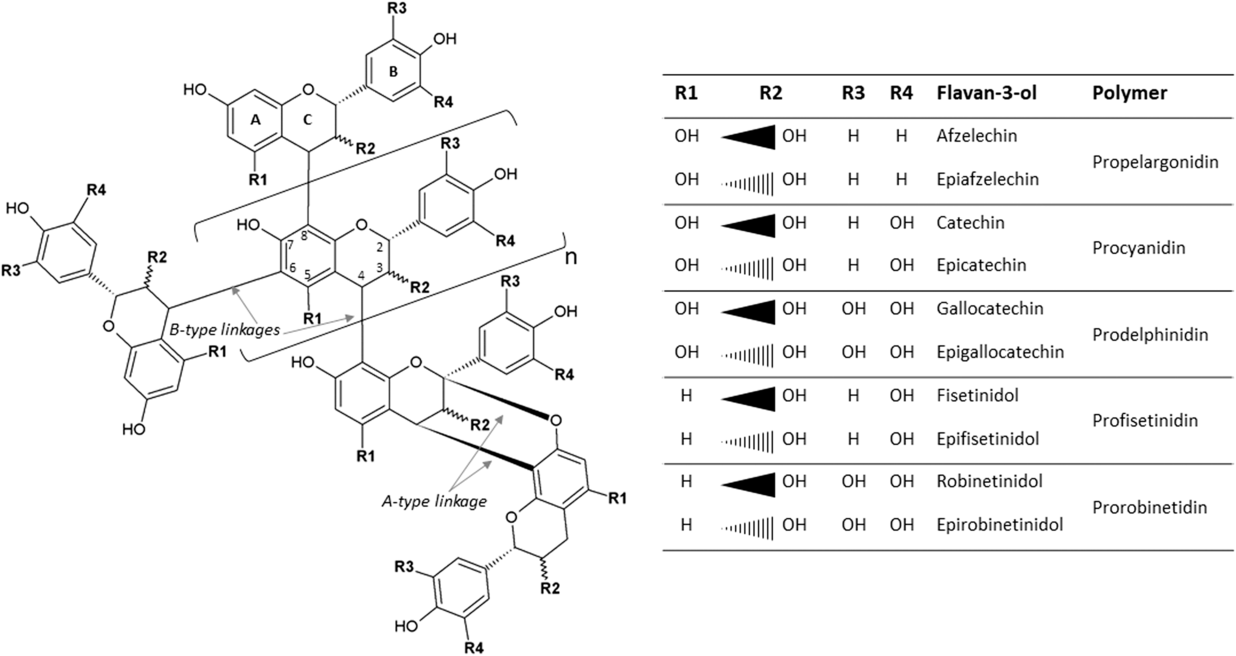
Figure 1 Condensed tannins consisting of typical flavan-3-ol subunits and two different linkage types.
The two main classes of HTs are the relatively rare gallotannins and the widespread ellagitannins. Simple gallic acid derivatives can be considered a third subclass of HTs (Salminen and Karonen, Reference Salminen and Karonen2011). Simple gallic acid derivatives and gallotannins contain gallic acid substituents that are esterified with a polyol residue, usually a D-glucose. However, glucitol, fructose, shikimic acid, xylose, hamamelose, saccharose, quercitol or quinic acid have also been identified as polyol residues in certain plants such as maple, chestnut, oak and witch hazel (Smeriglio et al., Reference Smeriglio, Barreca, Bellocco and Trombetta2017). In the first step of biosynthesis, galloylation reactions with 1-O-galloyl-β-D-glucose (β-glucogallin) yield di-, tri-, tetra-, and pentagalloylglucose (1,2,3,4,6-penta-O-galloyl-β-D-glucopyranose) that correspond to simple gallic acid derivatives (Figure 2). In the second step of galloylation, depsidic binding (meta- or para-depside) yields, among others, hexa-, hepta-, octa- and -galloylglucose; in fact, up to 12 units of gallic acids can be esterified on a single glucose moiety (Figure 2) (Sieniawska and Baj, Reference Sieniawska, Baj, Badal and Delgoda2017; Smeriglio et al., Reference Smeriglio, Barreca, Bellocco and Trombetta2017). Hydrolysis of gallotannins with strong acids generates the core polyol and gallic acid. Ellagitannins are produced through gallotannin oxidation by intermolecular carbon-carbon coupling between at least two galloyl units, forming hexahydroxydiphenoyl (HHDP; Figure 3a) or its derivatives, such as dehydrohexahydroxydiphenoyl units. In aqueous solutions, HHDP spontaneously lactonizes and releases ellagic acid (Figure 3b). Ellagitannins can be in monomeric, dimeric, oligomeric or C-glycosidic forms, and almost 500 different ellagitannin molecules have been isolated and identified to date (Smeriglio et al., Reference Smeriglio, Barreca, Bellocco and Trombetta2017). Complex tannins contain a carbon–carbon link between the central carbohydrate and a flavan-3-ol unit (e.g. epicatechin) plus galloyl and HHDP groups, such as camelliatannins (Figure 4; Hatano et al., Reference Hatano, Han, Taniguchi, Shingu, Okuda and Yoshida1995).
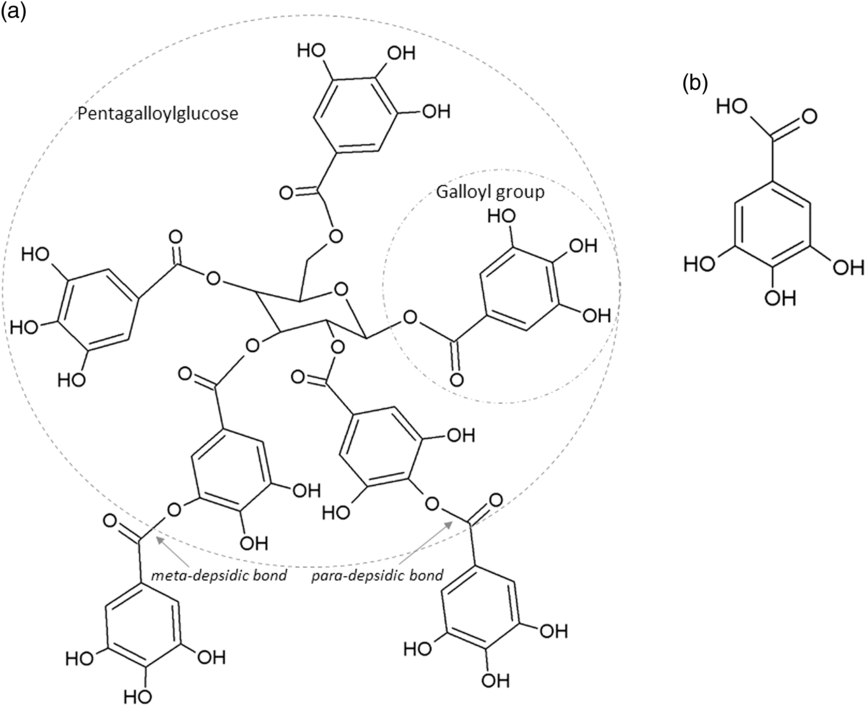
Figure 2 Chemical structure of: (a) a gallotannin molecule with depsidic bonds and (b) gallic acid.
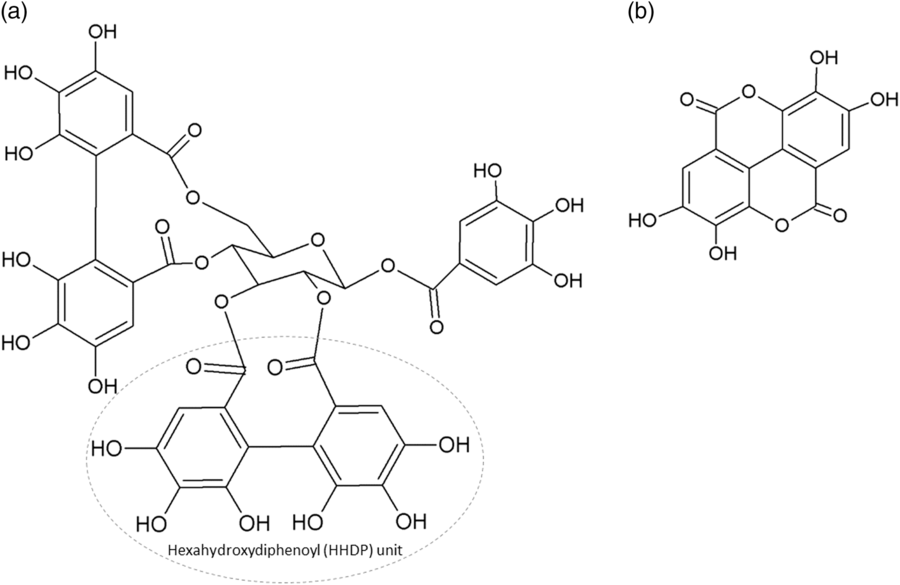
Figure 3 Chemical structure of: (a) an ellagitannin (casuarictin) with two hexahydroxydiphenoyl units and (b) ellagic acid.
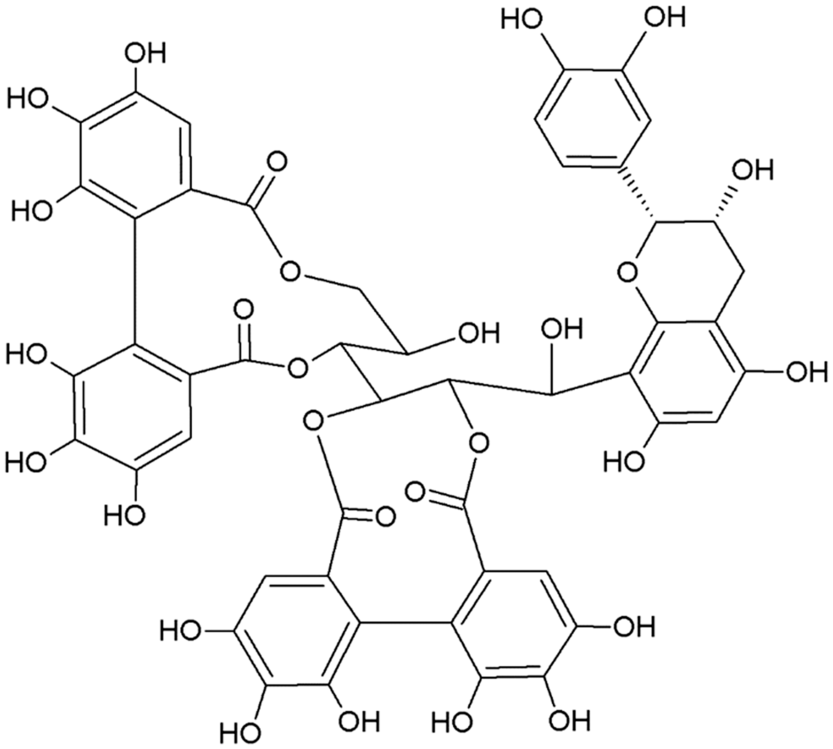
Figure 4 Chemical structure of camelliatannin E, a complex tannin.
Chemical and biological properties
Owing to their hydroxyl and phenolic groups, tannins interact in a number of ways with other molecules as reviewed by Girard (Reference Girard2016). These interactions are based on hydrogen bonding or hydrophobic interactions. They can also interact with molecules to form covalent bonds. Such bonds can occur under oxidative conditions, at high temperatures, at high pH, in ultraviolet radiation, in autoxidation or in the presence of catalytic enzymes, such as polyphenol oxidase, which produces reactive quinones (Barbehenn and Constabel, Reference Barbehenn and Constabel2011; Constabel et al., Reference Constabel, Yoshida and Walker2014).
Originally, tannins were used in tanning processes to convert animal hides into leather through the ability of tannins to adhere to proteins or, more precisely, to collagen. One of the main physico-chemical characteristics of tannins is their ability to interact strongly with proteins including enzymes. The maximum interaction between CTs and protein occurs when the solution pH is near the isoelectric pH of the protein; thus, for instance, greater affinities for CTs are reported at pH 4.9 for globular proteins with acidic isolectric points, whereas at pH 7.8, basic proteins have a higher affinity for CTs (Hagerman and Butler, Reference Hagerman and Butler1981). The interaction of tannins with salivary proteins and taste receptors, particularly bitter receptors, generates the astringent taste appreciated in wine and beer (Soares et al., Reference Soares, Kohl, Thalmann, Mateus, Meyerhof and De Freitas2013). In addition to proteins, various carbohydrates originating from the cell wall, such as pectin, cellulose or dietary fibers, can interact with tannins (Jakobek, Reference Jakobek2015). The detailed interactions between lipids and polyphenols (including tannins) have been described in a review of Jakobek (Reference Jakobek2015). In a previous study, He et al. (Reference He, Shi and Yao2006) showed a strong binding affinity between the gallic acid derivative pentagalloylglucose and phospholipids, which are constituents of the cell membranes. The interaction between HTs and phospholipids relies on the hydrophobic association between the galloyl groups of gallotannins and the hydrophobic hydrocarbon chains of phospholipids (He et al., Reference He, Shi and Yao2006; Yu et al., Reference Yu, Chu, Hagerman and Lorigan2011). Because of the orthohydroxyl groups present on their B-rings (catechol or pyrogallol), tannins can chelate and thereby sequester metal ions, particularly cations such as calcium, iron, magnesium, manganese and copper (Oladoja et al., Reference Oladoja, Alliu, Ofomaja and Unuabonah2011).
The aforementioned properties confer tannins to several biological applications, and their antiviral, insecticidal, nematicidal, antifungal, antibacterial and antioxidant activities have been well established (Barbehenn and Constabel, Reference Barbehenn and Constabel2011; Hoste et al., Reference Hoste, Torres-Acosta, Sandoval-Castro, Mueller-Harvey, Sotiraki, Louvandini, Thamsborg and Terrill2015; Smeriglio et al., Reference Smeriglio, Barreca, Bellocco and Trombetta2017). In particular, their potential antioxidant and anti-inflammatory effects on piglets would be interesting to help these animals to better cope with the ETEC-related diarrhea. However, our review only focuses on the effect of tannins on the pathogenic and commensal gut bacteria of piglets.
Tannin absorption and metabolism in the gastro-intestinal tract
Studies investigating the pharmacokinetics of tannins in animals are scarce. In the stomach, CTs remain relatively stable. The cleavage of CTs into monomers, such as flavan-3-ols, may occur, but it seems to be highly dependent on the solubility of CTs in this gastric juice and on the pH of the gastric juice (Zhang et al., Reference Zhang, Wang, Li, Ho, Li and Wan2016). Flavan-3-ols are often acylated by gallic acid (galloylation) and small amounts could be passively absorbed without being metabolized in the small intestine, along with CT dimers or trimers (Marín et al., Reference Marín, Miguélez, Villar and Lombó2015). Through the action of sulfotransferases, uridine-5’-diphosphate-glucuronosyl-transferases and catechol-O-methyltransferases, the main metabolites of flavan-3-ols or CT dimers in the plasma are sulfated, glucuronidated and methylated derivatives (Zhang et al., Reference Zhang, Wang, Li, Ho, Li and Wan2016; Mena et al., Reference Mena, Bresciani, Brindani, Ludwig, Pereira-Caro, Angelino, Llorach, Calani, Brighenti, Clifford, Gill, Crozier, Curti and Del Rio2019). However, oligomers larger than trimers and polymers cannot be absorbed via passive paracellular transport in their native form, and most ingested CTs reach the colon intact (Deprez et al., Reference Deprez, Mila, Huneau, Tome and Scalbert2001). The colonic microbiota catabolize CTs to produce hydroxyphenolic acids: phenylvaleric, phenylpropionic and phenylacetic acids, as well as phenylvalerolactones and benzoic acids (Marín et al., Reference Marín, Miguélez, Villar and Lombó2015). A part of these bioactive catabolites can be absorbed by the colonocytes. Subsequently, they are conjugated by specific hepatic enzymes to produce conjugated derivatives that are further eliminated in the urine (Mena et al., Reference Mena, Bresciani, Brindani, Ludwig, Pereira-Caro, Angelino, Llorach, Calani, Brighenti, Clifford, Gill, Crozier, Curti and Del Rio2019).
The polymers of HTs cannot be absorbed in their native form. Several types of bacteria, such as lactic acid bacteria, possess tannase activity (tannin acyl hydrolase), in which they hydrolyze the ester bonds of gallotannins and ellagitannins (although not the carbon–carbon bonds of ellagitannins; Mingshu et al., Reference Mingshu, Kai, Qiang and Dongying2006). Hydrolysis of ester and depside linkages of gallotannins by intestinal enzymes and colonic microbiota yields the core polyol (glucose) and gallic acid. The resultant aglycone (gallic acid) is metabolized to pyrogallol and phloroglucinol and, ultimately, to acetate and butyrate (Krumholz et al., Reference Krumholz, Crawford, Hemling and Bryant1987). Eubacterium oxidoreducans and Coprococcus sp., which are present in the rumen and the distal portion of the monogastric intestine, are involved in this reaction (Krumholz et al., Reference Krumholz, Crawford, Hemling and Bryant1987).
Due to their carbon–carbon bonds, ellagitannins are more difficult to degrade than gallotannins. Ellagitannins are resistant to the action of lactase-phlorizin hydrolase and β-glucosidase, present in the small intestine, and consequently are preferentially cleaved by colon microbiota (Marín et al., Reference Marín, Miguélez, Villar and Lombó2015). Hydrolysis of ellagitannins yields ellagic acid following lactonization. Whether ellagitannins can efficiently release ellagic acid under gastrointestinal physiological conditions without the involvement of specific gut microbiota remains unclear. In pigs fed acorns (rich in ellagitannins), ellagic acid has been shown to gradually be metabolized to urolithins throughout the intestine – to urolithins D and C in the jejunum and urolithins B and A in more distal parts of the intestinal tract (Espín et al., Reference Espín, Gonzalez-Barrio, Cerda, Lopez-Bote, Rey and Tomas-Barberan2007). Owing to the presence of ellagic acid metabolites in the bile and urine, as well as their absence in intestinal tissues, ellagic acid has also been suggested to be possibly absorbed in the stomach (Espín et al., Reference Espín, Gonzalez-Barrio, Cerda, Lopez-Bote, Rey and Tomas-Barberan2007).
Effects of tannins on bacteria
Pathogenesis of enterotoxigenic Escherichia coli infection
Diarrhea is a clinical symptom of an imbalance between absorption and secretion in the intestine, resulting in the hypersecretion of chloride and bicarbonate ions together with osmosis-driven water migration into the lumen. The main pathotype causing coliform PWD is ETEC. The pathogenicity of ETEC is due to their adhesins and enterotoxins, which impair enterocyte function and are primarily responsible for their virulence. After being ingested by ETEC-susceptible piglets, ETEC colonize the gastrointestinal tract by attachment to microvilli or mucus coating the epithelium via their fimbrial adhesins, enabling them to avoid elimination by peristalsis. Fimbriae are long, thin protein appendages present in high numbers (100 to 300 per bacterium) on the bacterial surface. The common types of fimbriae found in ETEC in piglets suffering from PWD are F18 and F4. The F18 fimbriae have two antigenic variants, F18ab and F18ac, whereas F4 fimbriae possess three antigenic variants, F4ab, F4ac and F4ad. These variants differ slightly in amino acid composition; variants F4ab and F4ad are made up of 264 amino acids, whereas variant F4ac is made up of 262 amino acids. Fimbriae primarily attach to specific receptors located on the apical side of enterocytes in the jejunum and ileum, but they may also bind to non-specific receptors in the mucus that coats the epithelium. The F4ad fimbriae are more likely to bind glycolipids, while the F18, F4ab and F4ac fimbriae preferentially bind to glycoproteins (Nagy and Fekete, Reference Nagy and Fekete2005). Once attached to the epithelium, ETEC replicate and secrete two types of enterotoxins, LT and ST ones, which are extracellular proteins and peptides. The pathogenesis of ETEC is summarized in Figure 5. The LT toxin has a high molecular weight (88 kDa, 343 amino acids), and its structure is similar to that of the Vibrio cholerae toxin. The LT toxin consists of six subunits: one A subunit with enzymatic activity and five B subunits (pentamer) involved in the adhesion of LT to the GM1 (monosialotetrahexoxylganglioside) receptor present on the surface of enterocytes. Once bound, LT is internalized by endocytosis and transported to the Golgi apparatus for disassembly. The A subunit migrates to the endoplasmic reticulum where it is cleaved to A1 (an NAD-dependent ADP-ribosyl transferase) and A2 (peptide) subunits. The activated A1 subunit transfers an ADP-ribosyl from NAD to the regulatory Gs protein α subunit located in the basolateral membrane. This results in the permanent activation of the adenylyl cyclase cascade and the increased intracellular concentrations of cyclic adenosine monophosphate (cAMP). The accumulation of cAMP activates protein kinase A (PKA), which phosphorylates the cystic fibrosis transmembrane regulator (CFTR) and a Na+/H+ exchanger (Dubreuil et al., Reference Dubreuil, Isaacson and Schifferli2016). This process leads to the secretion of chloride and carbonate ions and to the inhibition of Na+ reabsorption. Water is then osmotically drawn into the intestinal lumen, resulting in watery diarrhea (Dubreuil et al., Reference Dubreuil, Isaacson and Schifferli2016).
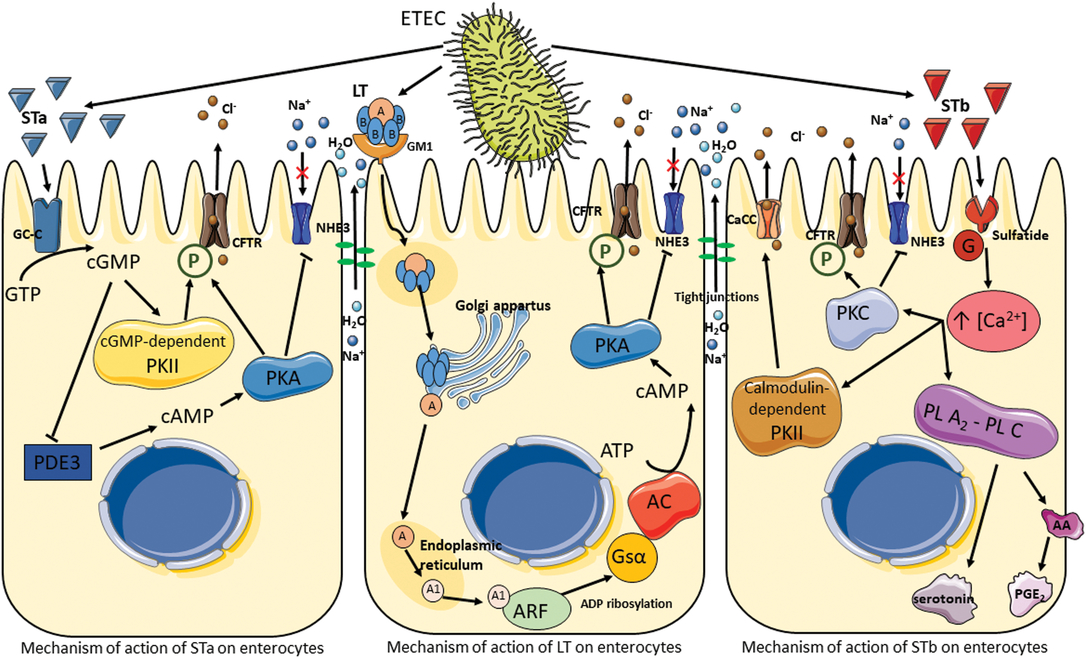
Figure 5 Pathogenesis of enterotoxigenic Escherichia coli. Signal cascade following toxin production by enterotoxigenic Escherichia coli, which results in the activation of ion channels and the disruption of tight junctions leading to electrolyte and water hypersecretion in the lumen. AA = arachidonic acid; AC = adenylate cyclase; ARF = ADP-ribosylation factor; CaCC = calcium-activated chloride channel; CFTR = cystic fibrosis transmembrane regulator; GC-C = guanylate cycles C; GM1 = monosialotetrahexoxylganglioside; G = protein; Gsα = Gs protein α; LT = heat-labile toxin; NHE3 = Na+/H+ exchanger 3; P = phosphorylation; PDE3 = phosphodiesterase 3; PGE2 = prostaglandin E2; PL A2-PL C = phospholipases A2 and C; PKA = protein kinase A; PKC = protein kinase C; PKII = protein kinase II; ST = heat-stable toxin.
Enterotoxigenic E. coli isolated from pigs produce two types of ST toxins: STa (18 amino acids and approximately 2 kDa) and STb (48 amino acids and 5.2 kDA). Both toxins remain active even after incubation at 100°C for 30 min. The STa mechanism is similar to that of LT. On the apical side of enterocytes, STa binds to guanylate cyclase-C, which synthesizes cyclic guanosine monophosphate (cGMP) from guanosine triphosphate. The accumulation of cGMP in the enterocytes has two effects: first, it activates a cGMP-dependent protein kinase II (PKII), inducing phosphorylation of the CFTR and secretion of chloride and carbonate ions; second, it inhibits phosphodiesterase 3, resulting in an increase in cAMP and the activation of PKA (Dubreuil et al., Reference Dubreuil, Isaacson and Schifferli2016). As in the LT signal cascade, CFTR and the Na+/H+ exchanger are phosphorylated, leading to a hypersecretion of electrolytes and water into the lumen.
The signal transduction pathway of STb does not involve cyclic nucleotides as secondary messengers. Research suggests that STb binds to a sulfatide receptor and is thereby internalized (Labrie et al., Reference Labrie, Harel and Dubreuil2002). The G protein cascade is then activated leading to an increase in the concentration of intracellular calcium, which activates a number of enzymes. The first is calmodulin-dependent PKII, which opens specific chloride channels and activates PKC; this in turn phosphorylates CFTR and inhibits Na+ uptake. The second and third activated enzymes are phospholipases A2 and C, which catalyze the release of arachidonic acid from membrane phospholipids as well as the formation of prostaglandin E2 and serotonin, known as secretory agents, from enterochromaffin cells (Peterson and Whipp, Reference Peterson and Whipp1995; Dubreuil et al., Reference Dubreuil, Isaacson and Schifferli2016). The release of these agents has been suggested to be a potential mechanism of effect for STb on the enteric nervous system (Peterson and Whipp, Reference Peterson and Whipp1995).
Finally, ETEC has been shown to induce pro-inflammatory responses with the production of cytokines in epithelial intestinal cells (Devriendt et al., Reference Devriendt, Stuyven, Verdonck, Goddeeris and Cox2010). However, the possible effects of tannins on inflammatory responses are not discussed in the present review (Williams et al., Reference Williams, Klaver, Laan, Ramsay, Fryganas, Difborg, Kringel, Reed, Mueller-Harvey, Skov, van Die and Thamsborg2017).
In vitro tannin inhibition of the growth of some bacteria
The bactericidal and bacteriostatic effects of CTs and HTs have been well documented (Smeriglio et al., Reference Smeriglio, Barreca, Bellocco and Trombetta2017). Table 1 presents tannin-containing plants with antimicrobial properties. Taguri et al. (Reference Taguri, Tanaka and Kouno2004) noted that of the 10 polyphenols they tested, the 2 flavan-3-ols, epigallocatechin and epigallocatechin gallate and the 2 tannins, castalagin and prodelphinidins, demonstrated the greatest antimicrobial activity against all strains of E. coli, particularly on ETEC strains. The A-type CTs from cranberries possess antibacterial activity against several pathogenic bacteria, including Helicobacter pylori, Salmonella, Staphylococcus aureus, E. coli, and Campylobacter (Côté et al., Reference Côté, Caillet, Doyon, Sylvain and Lacroix2010). The addition of 10 mg/ml HTs from chestnut wood extract had a slight bacteriostatic effect on the growth of ETEC F4ac, whereas 20 and 40 mg/ml exhibited marked bacteriostatic effects (Pradervand N, personal communication). Jelager et al. (Reference Jelager, Gurib-Fakim and Adsersen1998) observed that the antibacterial properties of tannins from Mauritian medicinal plants were lost when tannins were removed from the extract by precipitation with gelatin prior to testing. This finding confirmed that tannins were responsible for the observed antibacterial effects. Tannins may inhibit bacterial growth by destabilization and permeabilization of the cytoplasmic membrane and by inhibition of extracellular microbial enzymes, either by direct action against microbial metabolism and/or sequestration of the substrates required for microbial growth (Min et al., Reference Min, Pinchak, Merkel, Walker, Tomita and Anderson2008). For instance, tannins have been shown to chelate mineral macronutrients, such as iron or zinc, that are required for the growth of many bacterial species (Chung et al., Reference Chung, Wong, Wei, Huang and Lin1998). The addition of iron to the medium restored HT-inhibited bacterial growth (Engels et al., Reference Engels, Knödler, Zhao, Carle, Gänzle and Schieber2009).
Table 1 Tannin-containing plants with antimicrobial properties
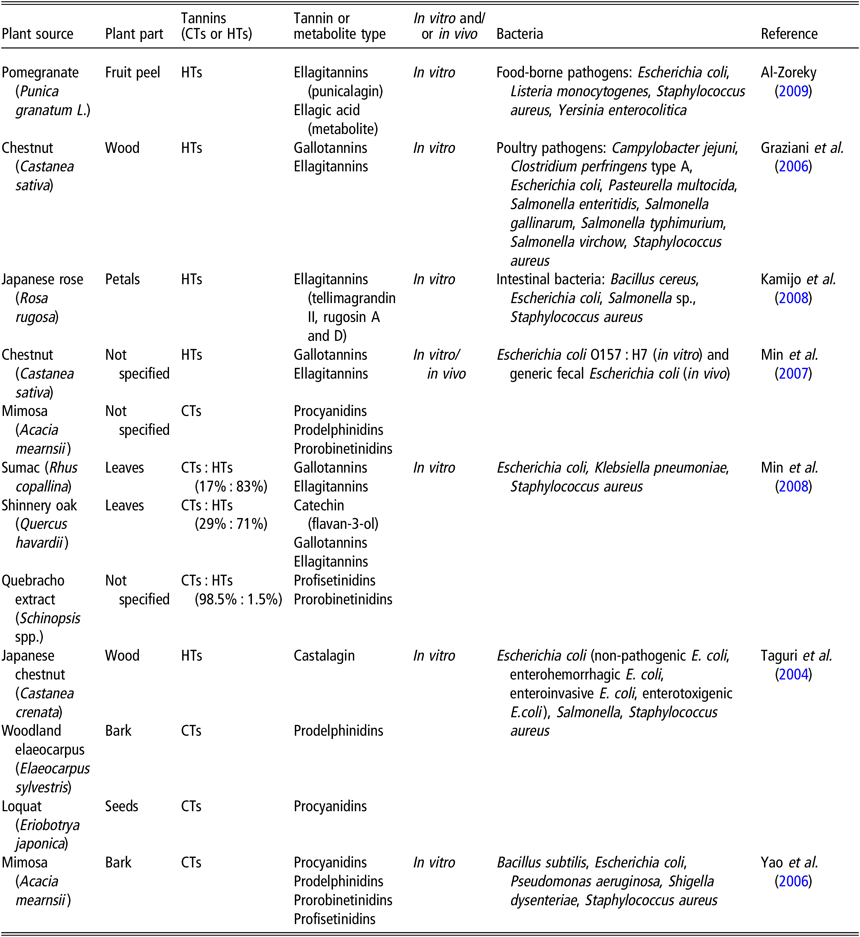
CTs = condensed tannins; HTs = hydrolyzable tannins.
Inhibition of bacterial adhesion to the intestinal epithelium and biofilm formation
Microorganism adhesion to the intestinal epithelium is a prerequisite step for bacterial colonization and biofilm formation. Cranberry juice containing A-type CTs has been reported to inhibit the adhesion of uropathogenic E. coli, which are responsible for urinary tract infections. The catabolites of cranberry CTs in the colon, the hydroxy-phenyl-γ-valerolactones, have been proposed as plausible candidates to exert anti-adhesive activity in the bladder (Mena et al., Reference Mena, Bresciani, Brindani, Ludwig, Pereira-Caro, Angelino, Llorach, Calani, Brighenti, Clifford, Gill, Crozier, Curti and Del Rio2019). Liu et al. (Reference Liu, Black, Caron and Camesano2006) noted that cranberry tannins decreased the adhesion forces between bacterial and epithelial cells and altered the conformation of surface macromolecules on E. coli, leading to a 60% decrease in the average P-fimbriae length (from 148 to 53 nm). A few studies focused on the effects of tannins on the intestinal epithelium adhesion of ETEC strains. Coddens et al. (Reference Coddens, Loos, Vanrompay, Remon and Cox2017) found that 10 µg of cranberry extract was sufficient to reduce the in vitro adhesion of verotoxigenic E. coli F18, whereas a greater amount (100 µg) was necessary to strongly inhibit ETEC F4 adhesion. These results were confirmed in ligated loop experiments on pigs in which the immunochemistry results clearly demonstrated that pre-incubation of F4 or F18 fimbriae with cranberry extract abolished the binding of fimbriae to the intestinal epithelial brush border. However, the authors demonstrated that this binding inhibition was not caused by an inhibition of bacterial growth. Similarly, Verhelst et al. (Reference Verhelst, Schroyen, Buys and Niewold2010) observed that at a concentration of 150 µg/ml, three tannin-rich extracts reduced ETEC F4ac binding to epithelial brush border tissues isolated from pigs. The three tannin-rich extracts contained pentagalloylglucose, a mixture of medium and high molecular weight HTs and procyanidins and flavan-3-ols of cocoa beans, respectively.
Some tannins can inhibit biofilm formation. Bacteria form biofilms following adhesion to a surface. Bacterial biofilm formation is now believed to play an important role in intestinal colonization (Hancock et al., Reference Hancock, Dahl and Klemm2010). Owing to its capacity to autoaggregate and form biofilms, a bacterial afimbrial adhesin involved in diffuse adherence (AIDA) associated with some diarrheagenic E. coli strains was shown to promote bacteria-to-bacteria adherence. A mutation of the genes coding for AIDA in an AIDA-positive ETEC resulted in an inability to aggregate and induce biofilms in the large intestine as well as to induce diarrhea (Ravi et al., Reference Ravi, Ngeleka, Kim, Gyles, Berthiaume, Mourez, Middleton and Simko2007). The biofilm facilitates the exchange of plasmids for horizontal gene transfer, concentrates bacterial enzymes that inactivate antibiotics and decreases the penetration of antimicrobials. Ellagitannins (mainly punicalagin) and ellagic acid from Punica granatum L. (pomegranate) extract inhibited the formation of biofilms in Staphylococcus aureus, methicillin-resistant S. aureus (MRSA), E. coli and Candida albicans (Bakkiyaraj et al., Reference Bakkiyaraj, Nandhini, Malathy and Pandian2013). In the same experiment, a minimum inhibitory concentration of 250 μg/ml of pomegranate extract was necessary to inhibit E. coli growth, but only 150 μg/ml of the same extract was necessary to fully inhibit biofilm formation. Similarly, a concentration of 8 mg/ml of gallic acid, a metabolite of gallotannin catabolism, inhibited E. coli biofilm formation at 25°C and 37°C (Shao et al., Reference Shao, Li, Li, Tang, Liu, Shi, Huang and Yang2015).
Inhibition of enterotoxin production and activities
Some tannins have the ability to inhibit bacterial enterotoxins or interrupt the signal transduction pathway of enterotoxins by inhibiting ionic channels. Elizondo et al. (Reference Elizondo, Mercado, Rabinovitz and Fernandez-Miyakawa2010) showed that CTs from quebracho, containing polymers of profisetinidins and prorobinetinidins, and HTs from chestnut, containing gallotannins and ellagitannins (mainly vescalagin and castalagin), were able to reduce the α toxin lecithinase activity and ϵ toxin cytotoxicity of C. perfringens. Similarly, 0.05% (v/v) of methanol extract from pomegranate extract containing ellagitannins (mainly punicalagin) and ellagic acid drastically reduced the production of staphylococcal enterotoxin A by S. aureus (Braga et al., Reference Braga, Shupp, Cummings, Jett, Takahashi, Carmo, Chartone-Souza and Nascimento2005). Similar results were observed for ETEC strains. Chen et al. (Reference Chen, Ho, Chang, Wu and Hsiang2006) showed that Galla Chinensis extract, which is rich in gallotannins and gallic acid, inhibited the binding of the B subunit of LT to its GM1 receptor, which may explain the antidiarrheal properties of this extract. Likewise, 750 μg/ml of two HT extracts, one containing the gallic acid derivative pentagalloylglucose and the other containing a mixture of medium and high molecular weight HTs, clearly reduced the binding affinity of LT for GM1, although the same concentration of a procyanidin CT extract had no inhibitory effect (Verhelst et al., Reference Verhelst, Schroyen, Buys and Niewold2010). However, the authors did not mention the tannin content of each extract. Certain specific tannins and flavan-3-ols are inhibitors of the ion channels involved in the development of diarrhea. The flavan-3-ols, epigallocatechin-gallate and epicatechin-gallate, from green tea (Camellia sinensis) and tannic acid (a mixture of gallotannins) inhibited calcium-activated Cl− channels by up to 50%, thereby reducing intestinal Cl− secretion. Epicatechin- or catechin-containing compounds are present in green tea and in grape seeds, but they have no documented effect on the aforementioned channels (Namkung et al., Reference Namkung, Thiagarajah, Phuan and Verkman2010). Schuier et al. (Reference Schuier, Sies, Illek and Fischer2005) demonstrated that procyanidins of cocoa beans partially blocked intestinal CFTR activity in a human colon epithelial cell line, which could explain their antisecretory properties.
Tannin effect on commensal microbiota and coliform diarrhea development
Several in vitro studies have shown that some tannins can have a negative effect on the growth of pathogenic bacteria and no or positive effects on the growth of commensal, non-pathogenic bacteria. For instance, ellagitannins isolated from Rosa rugosa petals (tellimagrandins II, rugosin D) showed antibacterial activities against E. coli, S. aureus, Bacillus cereus and Salmonella sp., but they had little or no effect against Bifidobacterium breve or Lactobacillus salivarius (Kamijo et al., Reference Kamijo, Kanazawa, Funaki, Nishizawa and Yamagishi2008). Similarly, tannic acid, some berries rich in CTs (mainly procyanidins) and gallotannins from Mangifera indica L. (mango) did not inhibit the growth of probiotic lactic acid bacteria, such as Bifidobacterium spp. and Lactobacillus spp. (Chung et al., Reference Chung, Wong, Wei, Huang and Lin1998; Puupponen‐Pimiä et al., Reference Puupponen‐Pimiä, Nohynek, Meier, Kähkönen, Heinonen, Hopia and Oksman‐Caldentey2001; Engels et al., Reference Engels, Knödler, Zhao, Carle, Gänzle and Schieber2009). The beneficial effects of tannins in reducing the growth of pathogenic bacteria and promoting that of beneficial bacteria have also been reported in in vivo trials (Biagi et al., Reference Biagi, Cipollini, Paulicks and Roth2010; Brus et al., Reference Brus, Dolinšek, Cencič and Škorjanc2013). Likely owing to their bactericidal and bacteriostatic properties, tannins have been found to modify the microbiota of pigs (Tretola M., personal communication). In a previous experiment, the addition of chestnut wood extract containing HTs to the feed of healthy pigs during fattening resulted in a reduction in the total E. coli number, an increase in the total lactic acid bacteria count and a greater average daily gain (ADG) (Brus et al., Reference Brus, Dolinšek, Cencič and Škorjanc2013). Supplementation with chestnut wood extract for 1 month following weaning tended to increase the viable counts of Lactobacilli in the jejunum, although the bacterial counts in the cecum were not affected (Biagi et al., Reference Biagi, Cipollini, Paulicks and Roth2010). Tannins were found to modify the microbiota of piglets artificially infected with ETEC. The inclusion of CT extract from grape seeds (10 g/kg in feed; B-type CT) and from cranberries (10 g/kg in feed + 1 g/l in water; A-type CT) in their rations significantly decreased ETEC F4 and verotoxigenic F18 E. coli shedding, respectively, and decreased the incidence of diarrhea (Verhelst et al., Reference Verhelst, Schroyen, Buys and Niewold2014; Coddens et al., Reference Coddens, Loos, Vanrompay, Remon and Cox2017). Although the severity of diarrhea in infected piglets was reduced upon 10 g/kg feed supplementation with chestnut wood extract rich in HTs, no reduction in ETEC F4 shedding in the feces was observed at 4 days post-infection compared with the infected piglets that did not receive HT supplementation (Girard et al., Reference Girard, Thanner, Pradervand, Hu, Ollagnier and Bee2018). Nevertheless, increasing the percentage of chestnut wood extract to 20 g/kg in the weaning diet of F4-susceptible piglets did result in lower ETEC F4 shedding in the feces at 3 days post-infection, a 40 g/day greater ADG and a marked decrease in the number of piglets with diarrhea (Girard M. and Hu D., unpublished observation). In addition, the inclusion of 20 g/kg of chestnut wood extract in the diets of piglets infected or not with ETEC F4 decreased the relative abundance of ETEC F4 and some Clostridium spp. in the jejunum without lowering the abundance of Lactobacillus spp. (Girard et al., Reference Girard, Pradervand, Silacci and Bee2019). In the aforementioned studies, the chestnut wood extract contained 45 mg/g gallotannins, 9 mg/g ellagitannins and 3.7 mg/g gallic acid.
Modifying factors of the bioactivity of tannins
The wide range of effects that tannins exert on pathogenic bacteria and their toxins is based on their ability to interact not only with proteins (including enzymes) but also with carbohydrates, lipids and metal ions. However, the bioactivity of tannins against bacteria varies according to the prevailing physico-chemical conditions and to factors related to bacteria and/or to the tannin molecules themselves.
Factors related to bacteria: type, concentration and time of exposure
An important factor is the target bacteria species. As previously mentioned, some CTs and HTs can inhibit the growth of some species but do not affect the growth of probiotic lactic acid bacteria (Kamijo et al., Reference Kamijo, Kanazawa, Funaki, Nishizawa and Yamagishi2008). Unlike other bacteria, some probiotic bacteria of the genera Bifidobacterium and Lactobacillus do not require heme-containing enzymes for metabolism and are not sensitive to the ion chelation induced by tannins. This may be why the growth of probiotic bacteria is not affected by tannins (Marín et al., Reference Marín, Miguélez, Villar and Lombó2015). In studies in which tannins influenced bacterial growth, researchers also demonstrated that Gram-positive bacteria, such as S. aureus, were more susceptible to tannins than Gram-negative bacteria, such as E. coli, were (Taguri et al., Reference Taguri, Tanaka and Kouno2004; Min et al., Reference Min, Pinchak, Merkel, Walker, Tomita and Anderson2008). The different susceptibilities between Gram-positive and Gram-negative bacteria might be related to their cell wall structures. Gram-positive bacteria have a single lipid bilayer surrounded by a thick porous layer of peptidoglycans, whereas Gram-negative bacteria have an inner lipid bilayer, a thin layer of peptidoglycans and an outer asymmetrical lipid bilayer on which lipopolysaccharides are anchored (Barer, Reference Barer, Greenwood, Barer, Slack and Irving2012). Compared with the double lipid bilayer, the single lipid bilayer might therefore be more easily destabilized by some tannins. The effects of tannins are directly associated with the concentration of bacteria and the duration of tannin exposure. Graziani et al. (Reference Graziani, Tosi and Denti2006) found an inhibitory capacity against 1.8 × 106 cfu/ml of E. coli using 1.5 g/kg of HTs from chestnut wood extract, but no inhibition was observed against 1.2 × 109 cfu/ml of E. coli at 1.0 and 2.5 g/kg of this same extract. Elizondo et al. (Reference Elizondo, Mercado, Rabinovitz and Fernandez-Miyakawa2010) observed a positive correlation between the time of exposure and the bactericidal effect of chestnut HTs against C. perfringens. Of note, the effectiveness of tannins in interfering with bacteria can be modified by tannin interaction with other compounds. Verhelst et al. (Reference Verhelst, Schroyen, Buys and Niewold2010) reported that the inclusion of bovine serum albumin (BSA) reversed the inhibitory effect of LT on its GM1 receptor, likely because tannins preferentially bind to BSA. Ropiak et al. (Reference Ropiak, Lachmann, Ramsay, Green and Mueller-Harvey2017) noted that the affinity of CTs for gelatin was greater than that for BSA, likely because CTs have high affinities for proline-rich proteins, such as gelatin that offers more binding sites (Hagerman and Butler, Reference Hagerman and Butler1981). Similarly, He et al. (Reference He, Shi and Yao2006) clearly demonstrated that pentagalloylglucose formed stronger hydrophobic associations with amino acids containing aromatic groups and aliphatic side chains. These findings reveal that the extent of tannin-protein interaction strongly depends on the amino acid profile.
Associated factors: tannin dose, type and chemical structure
The beneficial effects of tannins depend primarily on the dose of tannins in the media or the diet. Both CTs from quebracho and HTs from chestnut inhibited the growth rate and toxic effects of C. perfringens in a dose-dependent manner (Elizondo et al., Reference Elizondo, Mercado, Rabinovitz and Fernandez-Miyakawa2010). Chen et al. (Reference Chen, Ho, Chang, Wu and Hsiang2006) also presented a clear dose-dependent inhibition of LT binding to GM1 with increasing concentrations of Galla Chinensis extract containing gallotannins. However, several studies highlighted the fact that the type of tannins is also important (Digrak et al., Reference Digrak, Alma, Ilçim and Sen1999; Elizondo et al., Reference Elizondo, Mercado, Rabinovitz and Fernandez-Miyakawa2010). Elizondo et al. (Reference Elizondo, Mercado, Rabinovitz and Fernandez-Miyakawa2010) found that 8 mg/ml of HTs of chestnut wood extract (gallotannins and ellagitannins) had strong bactericidal properties, whereas 7.5 mg/ml of CTs from quebracho, containing polymers of prorobinetinidins and profisetinidins, had no effect. Despite a lower tannin content, CTs from Acacia mollissima (mimosa bark), containing polymers of prorobinetinidins and profisetinidins, possessed greater antibacterial activity than did ellagitannins (mainly castalagin and vescalagin) from the gallnuts of Quercus macrolepis (valonia oak) and gallotannins from Quercus infectoria (Digrak et al., Reference Digrak, Alma, Ilçim and Sen1999). At the same concentration, Yu et al. (Reference Yu, Chu, Hagerman and Lorigan2011) showed that pentagalloylglucose, a nonpolar gallic acid derivative, disordered the acyl chains of the lipid bilayers compared with a very polar CT trimer of catechins. The authors suggested that the polarity of the tannin was inversely related to the strength of interaction.
The method of tannin extraction can also affect the bioactivity of tannins because different solvents extract different types of tannin molecules (Chen et al., Reference Chen, Ho, Chang, Wu and Hsiang2006; Yao et al., Reference Yao, He, Ying Jia and Shi2006; Unaeze et al., Reference Unaeze, Ilo, Egwuatu, Orabueze and Obi2017). In a previous experiment, Chen et al. (Reference Chen, Ho, Chang, Wu and Hsiang2006) compared the ability of several soluble fractions of Galla Chinensis extract to inhibit the binding of LT to GM1. At 500 μg/ml, an ethyl acetate soluble fraction of Galla Chinensis extract completely inhibited the binding of LT to GM1, whereas a 50% inhibition was observed for the butan-1-ol fraction, and no inhibition was found for aqueous soluble fractions. These differences might be directly associated with the chemical properties of tannins, such as the molecular weight of the tannin molecule, or the degree of galloylation of the gallotannin polymers, and tannin composition. Yao et al. (Reference Yao, He, Ying Jia and Shi2006) confirmed the importance of molecular weight in relation to biological activity (interaction with proteins, enzyme inhibition and antimicrobial potency) by comparing different fractions of CTs from Acacia mearnsii (black wattle). The average molecular weights of water, ethyl acetate and ether extracts were 2050, 980 and 440 Da, respectively. The authors found that water fraction with high molecular weight had the strongest capacity to interact with proteins, whereas the ether extract with low molecular weight displayed greater antibacterial potency. Ethyl acetate extract, which was of medium molecular weight, had both a strong capacity to inhibit enzyme activity and strong antibacterial properties. Baert et al. (Reference Baert, Pellikaan, Karonen and Salminen2016) also demonstrated that tellimagrandin I-based oligomeric ellagitannins isolated from Epilobium angustifolium (rosebay willow herb) flowers decreased gas production and total volatile fatty acid concentration proportionately to their degree of oligomerization. Because gas, such as methane, and volatile fatty acids are produced by the rumen microbiota, the authors hypothesized that these ellagitannins may have a direct inhibition of the methanogenic microbiota and/or could bind to dietary fibers, the substrate required for gas production. Similar conclusions were drawn by Ropiak et al. (Reference Ropiak, Lachmann, Ramsay, Green and Mueller-Harvey2017), who showed that the greater the mean degree of polymerization or the average molecular weight, the fewer the moles of CTs are needed to precipitate the same amount of BSA or gelatin. Therefore, CT size is an important factor to consider in CT-protein interaction. In the case of ETEC-related diarrhea, testing whether small CTs would differently affect ETEC growth, biofilm formation, ETEC adhesion to the mucosa and ST and LT enterotoxin activities compared with larger CTs would be worthwhile. Finally, the chemical composition of tannins is another important component relating to tannin bioactivity. Among the diverse family of tannins, each plant has a unique chemical composition. The CTs from Schinopsis lorentzii (quebracho) and Acacia mollissima (mimosa bark) are polymers with different profisetinidin : prorobinetinidin ratios, whereas the CTs from temperate forage legumes are polymers with different percentages of procyanidins and prodelphinidins. Prodelphinidins have more hydroxyl groups than procyanidins, which generally indicates greater reactivity toward protein. Greater molar proportions of prodelphinidins have been shown to decrease the apparent α-helix content and increase the apparent β-sheet content of BSA, which indicates a conformational change in the tertiary structure of the protein (Ropiak et al., Reference Ropiak, Lachmann, Ramsay, Green and Mueller-Harvey2017). The ability of such tannins to affect protein structure could be of interest to inactivate some proteins involved in ETEC pathogenesis, such as fimbriae, enterotoxins (ST and LT) and their respective receptors in the enterocytes. The antimicrobial activity of flavan-3-ols and CTs can be modified by the presence of galloyl groups as opposed to hydroxyl groups at the C3 position of the C ring (Taguri et al., Reference Taguri, Tanaka and Kouno2004). Similarly, comparing the binding properties of gallotannins and ellagitannins, Deaville et al. (Reference Deaville, Green, Mueller-Harvey, Willoughby and Frazier2007) highlighted the importance of the conformational flexibility of tannin molecules. Their HHDP groups render ellagitannins less flexible than gallotannins. The authors demonstrated that gallotannins and pentagalloylglucose bind with equal strength to gelatin and BSA, whereas less-flexible ellagitannins bind more strongly to flexible proteins such as gelatin, and weakly to BSA. Therefore, gallotannins may be more interesting than ellagitannins to interact with a larger range of proteins involved in ETEC pathogenicity, while ensuring that the dose is not deleterious to the animal ingesting them.
The aforementioned results demonstrated that differences in the chemical structure of tannins, such as tannin size (degree of polymerization, galloylation or oligomerization) and composition, lead to differences in tannin properties, such as the flexibility, the hydrophobicity and the polarity of tannin molecules. In turn, this results in varying affinities for proteins and lipids, which ultimately disrupt ETEC pathogenicity. Moreover, owing to the broad diversity of tannins, concluding which ones are the most promising in reducing the development of coliform PWD is very difficult at present because both types of tannins, CTs and HTs, can affect ETEC pathogenicity. In addition, numerous in vivo experiments use commercial extracts that are mixtures of tannins, and very often, no information about the detailed tannin composition is given. Finding a type of tannin or a combination of tannins that would affect, at the same time, bacterial growth, bacterial attachment to the mucosa and enterotoxin activities introduces new challenges. Designing innovative experiments that can establish the types of tannins or tannin-containing feeds that are most effective and at which dose would help to tackle these challenges. This can be achieved through a careful choice of tannin-containing plants or the selection or breeding of the required plant models. Nevertheless, some gallotannins or gallic acid derivatives, such as pentagalloylglucose (940 Da), seem promising in concomitantly disrupting lipid membranes and in interacting strongly with different proteins. Their ability to reduce the binding of ETEC to brush borders and to disrupt the binding between the LT toxin and its receptor GM1 has proven this (Verhelst et al., Reference Verhelst, Schroyen, Buys and Niewold2010).
Conclusion
Tannins offer great potential for the prevention of microbial infections and the reduction of coliform PWD. With their numerous hydroxyl and phenolic groups, tannins exhibit several biological properties with a wide range of effects on bacteria and on enterotoxin production and their activities. They directly act on pathogenic bacteria to inhibit or slow down growth by preventing bacterial adhesion to the intestinal epithelium and by inhibiting the bacterial enterotoxins and channels involved in the secretion of electrolytes and water into the lumen. In addition, some tannins seem to have prebiotic effects in the gut, together with antioxidant and anti-inflammatory effects, which were not discussed in the present review but may help piglets better cope with ETEC-related diarrhea. In vitro results are supported by in vivo experiments showing that the inclusion of tannins in the diet can decrease the severity and occurrence of diarrhea. This can engender improved health status and growth performance, as well as promote pig welfare via improved appetite. Tannins may counteract the intestinal dysbiosis that occurs at weaning, promoting and maintaining optimal gut health.
Disparities in terms of efficacy and specificity exist among the different types of tannins present in a plant or extract, as well as differences attributed to their concentration and chemical structure. Further investigations are needed to determine the optimal combinations of tannins and the most suitable and cost-effective manner with which to deliver them. Certain discrepancies between in vitro and in vivo results could be explained by the metabolism of tannins along the intestinal tract. Whether and which tannins and their metabolites remain active in the intestine warrant further research. Their antibacterial properties and the possibility for bacteria to develop resistances to tannins should be investigated, as well as how this risk could be minimized.
Acknowledgements
The authors gratefully acknowledge the Pig Commission of the European Association of Animal Production (EAAP) for the opportunity to present this review. This work refers in part to the literature review of the doctoral thesis of Marion Girard funded by the European Marie Curie Initial Training Network (‘LegumePlus’; PITNGA-2011-289377).
Declaration of interest
The authors declare that they have no competing interests.
Ethics statement
None.
Software and data repository resources
None.