NOMENCLATURE
- BPF
-
Blade Passing Frequency
- LAmax
-
A-weighted noise level, maximum value
- rpm
-
rounds-per-minute
- VTOL
-
Vertical Take-off and Landing
- CP
-
power coefficient
- f
-
frequency, Hz
- n
-
number of propeller blades
- r
-
propeller radius, m
- V
-
flight speed, m/s
- W
-
weight, kg
- z
-
flight altitude, m
-
$\eta$
-
propulsive efficiency; overall efficiency
-
$\theta$
-
propeller pitch angle, degrees/radians
-
$\Omega$
-
angular speed, rad/s
1.0 INTRODUCTION
The archives of the headquarters at the Royal Air Force Club in central London contain a collection of paintings from the golden age of aviation, when everything seemed possible. Aviators soared into the clouds on vehicles which today we would call unsafe, unreliable, and noisy. Today there is a can-do attitude that reminds the early the 20th century, when colorfully posters advertised aviation events. The creative arts are back in fashion with modern-day William Turners showing paintings of new flying vehicles in the evening glow—often prototypes superimposed to city scapes to create the illusions that those vehicles are actually flying. Our knowledge of these new systems is limited, but our optimism is unbounded. The allure of technology presumably stems from our ability to capitalise on large data sets to simulate complex scenarios. Network technologies offer high speed, high bandwidth streaming of information, using affordable, and readily available hardware and communication protocols and can be programmed to take rapid decisions where where humans would struggle.
A spectacular success has been achieved in integrating off-the-shelf technologies to produce sub-scale flight systems (drones) which, supported by the availability of sensors and communication systems, have generated both commercial and military interest. To-date, over 100 prototype electrically powered vertical-lift vehicles (eVTOL) have been proposed, only a handful of which have demonstrated some flight capability. A database is held by the Vertical Flight Society(Reference Hirschberg1).
One cannot fail to notice that all proposed designs use rotors; this is due to the efficiency of low-speed rotary wings, and the need for almost vertical take-off and landing.
The set of available tools lacks a deep understanding of the fundamental flight physics, with several misconceptions still dominating. This includes new problems that simply do not occur at the full-scale of flight, as well as integration issues arising from the use of technologies originally not intended to perform as an integrated flight platform. However, they aim to deliver air mobility systems with ambitious plans, such as making personal air vehicles widely available. There are scenarios of air vehicles of all sizes for a variety of missions in free flight. This is a dystopic future, the future envisioned by science fiction films; this is a future where the sky would be free, personal transport would be inexpensive, and flight would be safe. This is demonstrated as a fallacy.
There are compelling differences in our current understanding between these new systems and conventional rotorcraft. Thus, we need to separate these two strands of research but equally take advantage of what we already know to create the knowledge base. The consensus appears to be the need to increase power densities to a level comparable to those of aviation fuel; this achievement is unrealistic and far into the future. Alternative solutions do exist. Thus, the question to answer is:
How we can harness electrical power, alongside new vehicle architectures, to enable the development of new flight systems capable of delivering new services that can be measured with new metrics?
Several fundamental aspects of urban air vehicles have not been fully understood; their limitations have been overlooked, and the risks have been down-played. In a recent piece of news, Über conceded that helicopters are the real flying cars, for now, and started using direct helicopter services between city centres and major airports. This concession highlights the major problem with the prototypes being proposed: They are at a very early stage, not properly costed, not certifiable, may have unspecified environmental impact, and pose enormous risks to passengers and ground infrastructure—all this in absence of an air traffic control system that can monitor low-flying vehicles. There is no recognised optimal solution, although a number of prototypes exist, each using multiple rotors, and no infrastructure that can support them. Duffy et al(Reference Duffy, Wakayama and Hupp2) in their analysis include a comparison with the Robinson R44 for sizing purposes, which is very interesting, because it highlights more clearly where the design constraints are and how we must depart from the conventional helicopter configuration in order to take advantage of electric propulsion.
Several novel technologies are being presented at the same time, with little knowledge of integration between electrical systems, power plants, composite materials and heat dissipation issues. These are likely to lead to systems that have lower technology readiness than the core components, and will require considerable time to be solved. We are moving in an incrementally revolutionary direction with bumps and setbacks along the way.
2.0 INTERNATIONAL CONTEXT
In November 2018, NASA launched an Urban Air Mobility Challenge. In this process a number of companies were engaged in technology forecasting. After interviews with over 100 experts, it was concluded,1 that for this urban air mobility to be viable it is necessary to address technical, physical, operations and integration challenges of a highly interdependent system-of-systems.
There has been a quick growth in technical papers starting in 2018, to ride this challenge(Reference Thipphavong3,Reference Patterson, Antcliff and Kohlman4) , also on the back of a white paper published by Über(5) and advocates of Aviation 4.0.2 Since then, a number of white papers, opinion papers, and forecast assessments have been multiplying, most recently Ref.(Reference Dubno6). Technology forecast is split among data services and goods, including the delivery of parcels, data, aerial observation or monitoring for several purposes: telecommunications, security, scientific research and medical services.
The issues of public acceptance and regulatory constraints have been highlighted as potential obstacles, with research now converging toward noise emissions, both internal and external, as a key road-block(Reference Alexander, Whelchel, Intaratep and Trani7,Reference Edwards and Price8) . In a subsequent analysis, several technologies have been identified as having low levels of maturity, including battery/power, navigation, surveillance, airspace design, flight procedures, etc. Advanced flight platforms at the small scale (
$\sim$
10cm) have been shown by some technology companies, who effectively demonstrated that the conventional configuration of single rotor with a tail rotor is both scalable and efficient. Therefore, why proposing rotor systems that contain multiple rotors?—In fact, some of the full-scale prototypes being proposed as personal air transport have no different function than helicopters, but they add complexity through the power system and the distributed propulsion(Reference Kim, Perry and Ansell9), which are likely to be more costly than conventional helicopters. Scaling constraints have been identified by Vascik & Hansmann(Reference Vascik and Hansman10), who also demonstrated that ground infrastructure, air traffic control and noise are three key limiters to the development of urban air systems. These limiters are not investigated in some conceptual designs (for example, Refs(Reference Johnson and Silva12,Reference Vegh, Botero, Clark, Smart and Alonso13,Reference Kohlman and Patterson14)) that instead address a broad technology challenge and highlight the difficulties of developing new concepts from a clean sheet.
In November 2018, NASA launched an Urban Air Mobility Challenge. In this process a number of companies were engaged in technology forecasting. After interviews with over 100 experts, it was concluded,3 that for this urban air mobility to be viable it is necessary to address technical, physical, operations and integration challenges of a highly interdependent system-of-systems.
There has been a quick growth in technical papers starting in 2018, to ride this challenge(Reference Thipphavong3,Reference Patterson, Antcliff and Kohlman4) , also on the back of a white paper published by Über(5) and advocates of Aviation 4.0.4 Since then, a number of white papers, opinion papers, and forecast assessments have been multiplying, most recently Ref.(Reference Dubno6). Technology forecast is split among data services and goods, including the delivery of parcels, data, aerial observation or monitoring for several purposes: telecommunications, security, scientific research and medical services.
The issues of public acceptance and regulatory constraints have been highlighted as potential obstacles, with research now converging toward noise emissions, both internal and external, as a key road-block(Reference Alexander, Whelchel, Intaratep and Trani7,Reference Edwards and Price8) . In a subsequent analysis, several technologies have been identified as having low levels of maturity, including battery/power, navigation, surveillance, airspace design, flight procedures, etc. Advanced flight platforms at the small scale (
$\sim$
10cm) have been shown by some technology companies, who effectively demonstrated that the conventional configuration of single rotor with a tail rotor is both scalable and efficient. Therefore, why proposing rotor systems that contain multiple rotors?—In fact, some of the full-scale prototypes being proposed as personal air transport have no different function than helicopters, but they add complexity through the power system and the distributed propulsion(Reference Kim, Perry and Ansell9), which are likely to be more costly than conventional helicopters. Scaling constraints have been identified by Vascik & Hansmann(Reference Vascik and Hansman10), who also demonstrated that ground infrastructure, air traffic control and noise are three key limiters to the development of urban air systems. These limiters are not investigated in some conceptual designs (for example, Refs(Reference Silva, Johnson, Solis, Antcliff and Patterson11,Reference Johnson and Silva12,Reference Vegh, Botero, Clark, Smart and Alonso13)) that instead address a broad technology challenge and highlight the difficulties of developing new concepts from a clean sheet.
3.0 IDENTIFICATION OF TECHNOLOGY GAPS
We have identified several gaps which arise from a number of important misconceptions that prevent technological progress. These arise from selecting sub-set of facts and ignoring others, in a hurry to be first to succeed, or because of genuine lack of knowledge.
Fallacy #1: Urban air systems are no different than existing helicopters
Many design concepts appear to be retrofit exercises, whereby off-the-shelf propellers are coupled to electric motors and distributed around the airframe in large numbers, and with movable axes. These retrofits ignore several fundamental problems, among which is the non-exhaustive list below:
(1) Discipline experts produce local optima following their own metrics, regardless of the compromises required to guarantee multi-disciplinary optimal solutions; (2) propeller propulsion is prone to loss of efficiency due to inappropriate wing-nacelle-stator integration (wing circulation impact, blade-vortex interaction on hard surfaces and among rotors); (3) distribution of several smaller propellers rather than a smaller number of larger propellers may have an advantage from the point of view of electrical power distribution (though this is still unclear). However, it is a fundamental fact of flow physics that it is more efficient to propel a mass of air at low speed through a large stream tube; (4) rotor interference, albeit occasionally beneficial, is a source of unwanted noise; (5) there is lack of consideration of high disk loadings, danger zones in the flight conversion corridor, thrust vectoring issues, recovery strategies in case of power supply failure or load imbalance; (6) what flight envelope is to be expected?
A database compiled by the Vertical Flight Society indicates that only a minority of vehicles has demonstrated some flight capability; of these, payload capacity does not exceed 300kg (3 passengers) and endurance is limited to 1 hour at most, Fig. 1.

Figure 1. eVTOL data from both flying and non-flying prototypes. Scatter size proportional to battery power, as indicated. Data source: Hirschberg(Reference Hirschberg1), Vertical Flight Society.
Many of the assessments available in the published literature concede that range is interpreted as flight on a straight line. Even then, there are proposals for last-mile delivery, with radius of action not exceeding a metropolitan area. Contributions in this area include Refs(Reference Kohlman and Patterson14,Reference Bosson and Lauderdale15). However, this is unlikely to happen if we apply constraints from the urban areas, no-fly zones over public infrastructure (high-rise buildings, hospitals, schools, congested roads). Depending on the infrastructure we are willing to put in place to support urban air mobility, the configuration of the optimal aerial vehicle may be different.
There is no experience with passenger comfort, because there is no real flight demonstration available to gather data for handling qualities. Thus, we are unable to assess issues such as the effects of vehicle motion on passenger well-being (g-accelerations), effects of turbulence and rapid manoeuvres. Recently, it has been established that passenger acceptance is not guaranteed(Reference Edwards and Price8).
Fallacy #2: Urban air vehicles (manned or unmanned) can fly anywhere
Surely not! It is abundantly clear that several constraints exist to the development of urban air mobility, in spite of claims of “a new generation of increasingly cleaner and quieter aircraft capable of transporting anything…” (quoted from the website of a major aero-engines manufacturer, but reported in other studies). There are other examples in the technical literature claiming that eVTOL have the “potential of enabling point-to-point air travel in congested cities”(Reference Ha, Lee and Hwang16). Yet, this type of services is already available by helicopter, with several safety limitations, among which the rotor downwash is an important example. There is a further emphasis on the use of eVTOL air taxis to “alleviate transportation congestion” (for example, Refs(Reference Pradeep and Wei17,Reference Verma, Keeler, Edwards and Dulchinos18)), although it is not made clear what amount of eVTOL taxi capacity is required to bring about this alleviation.
In the case of the eVTOL, any vehicle with multiple rotors is likely to have a high disk loading (thrust/disk-area), which will force operations away from unprepared surfaces (not anywhere), away from ground personnel (away from people) and away from ground obstacles, which cause turbulence amplification and noise emissions at high frequencies.
Disk loading can be reduced by spreading the vertical lift among a large number of rotors having a total disk area larger than a conventional helicopter. If the overall diameter is to be constrained to that of a conventional helicopter rotor (a fair assumption), a quad-rotor would have a considerably lower swept area and thus a higher average downwash per rotor. Reasonably, a quad-rotor can be fitted within a single-rotor diameter with a diameter ratio of 0.4 (two rotors side-by-side taking 80% of the overall diameter; the theoretical limit that avoids rotor overlap is
$\sim$
0.65). This implies that the total area swept is only 64% of the original one, and the mean downwash of each rotor would increase by
$0.25 \div 0.4^2= 1.25$
, or 25%. A similar analysis applied to the Volocopter 2X—following the manufacturer’s data on propellers and weight—indicates a propeller downwash that is
$\sim$
30% higher than an equivalent single rotor (diameter of 9.15m). Higher downwash can be achieved with higher tip speed, and this would have implications on the overall noise emission.
One positive aspect that must be considered is the quietness of electrical machines. VSTOL concepts would alleviate failure events at take-off and landing, but would require new ground infrastructure; this is unlikely to be forthcoming. Vascik & Hansmann(Reference Vascik and Hansman10), demonstrated that ground infrastructure, air traffic control and noise are three key limiters to the development of systems for urban air mobility.
The most important market is likely to be the large conurbations of North America and parts of Asia, where urban sprawl often involves long commuting distances—less likely in Europe, where space is at a premium, the possibilities of adding ground infrastructure rather limited (in the UK, only three hospitals have a roof heliport), the financial benefits are dubious and public acceptance is very limited, not least because helicopter ownership is viewed as the the privilege of high net-worth individuals.
Examples shown in the literature refer to the “Los Angeles area”, or Dallas or San Francisco, which are not representative of the world at large(Reference Ha, Lee and Hwang16,Reference Verma, Keeler, Edwards and Dulchinos18) , since these large conurbations do not have a network of public transportation infrastructure that competes with the aircraft. In other reports we read that “consumers” would use a ride-sharing operation “to call VTOL to their desired pickup location and specify drop-off destinations at rooftops throughout a given city”.5
The number of certified heliports in the UK and Ireland is about 1,000, and the distance among some of these is larger than the range predicted for most eVTOL, making them virtually unreachable. The nearest heliports to the authors are 6 miles and 1.7 miles, respectively, a relatively short distance, but not sufficient to hop on and off an eVTOL. Some discussions of optimal heliport placement(Reference Daskilewicz, German, Warren, Garrow, Boddupalli and Douthat19) are not realistic, although the mathematical argument is impeccable.
Thus, the scenario recalled neglects the very important problem of integration between the vehicle and the city infrastructure, which would require large investments and new health and safety regulations. In other words, the development of the flight platform would be dependent on the urban support network, and therefore it calls for a system-of-systems integration.
In other cases, for example Refs(Reference Warren, Garbo, Herniczek, Hamilton and German20,Reference Duffy, Wakayama, Hupp, Lacy and Stauffer21), some items of direct operating costs are missing from the accountancy, not least the acquisition costs, the pilot training requirements, leading to a profitability that is optimistic, when compared to a conventional helicopter (the work of Duffy et al(Reference Duffy, Wakayama, Hupp, Lacy and Stauffer21) indicates that depreciation of eVTOL may be higher than the conventional helicopter).
Rotorcraft pilot training costs are known to be high, and they will not disappear with the future eVTOL. With regards to the acquisition costs, these cannot be established with any certainty, unless the manufacturing infrastructure is developed, and the order numbers are confirmed firmly. Forecasts of future sales for a product that does not exist are notoriously unreliable.
Fallacy #3: Urban air vehicles are not a threat to the environment
The take-off of an urban air vehicle does not start like a Handel concerto: The humming noise of several propellers is unbearable. Key environmental metrics such as community noise, energy use, life-cycle greenhouse gas emissions, aerial threats, and public space trespassing, have not been critically evaluated in this context. No environmental benefits have been demonstrated anywhere. The authors have witnessed a case, from working on an engineering project, of a drone design that produced 110–130dB of noise from 1.8m diameter propellers and could not be flown (for reference, peak noise level from Concorde at take-off was
$\sim$
115dB). The advantage of quiet electrical propulsion is unhelpful.
With regards to noise, there is research that is based on experimental measurements of specific drones(Reference Alexander, Whelchel, Intaratep and Trani7,Reference Tinney and M22) , with results that cannot be generalised and offer limited insight. Extensive analysis exists already on helicopter operations(Reference Leverton23,Reference Basner, Babisch, Davis, Brink, Clark, Janssen and Stansfeld24) . One key difference is that gas turbine engines noise has a different frequency content from an electrical machine. In our case, noise is essentially limited to the rotors and their mutual interference, with noise from electrical systems presumably negligible. There is no research on cabin/indoor noise, which would affect directly passengers. This is clearly rather difficult in the absence of functioning vehicles, any flight experience, or any empirical data and measurements to help with the assessment of flight comfort.
Why would multiple rotors produce less noise than a single one? No proof has been shown that this is the case. Figure 2 compares the acoustic emissions of a single propeller in slow axial flight versus a scaled propeller. The graph shows the noise in the frequency domain where the frequencies are limited to 1/3 octave bands. The method used is a modification of Hanson & Parzych(Reference Hanson and Parzych25), with aerofoil broadband noise calculated with an implementation of Amiet’s aerofoil broadband noise model(Reference Amiet26), as used in previous work by one of the authors(Reference Filippone27,Reference Filippone and Mohammed-Kassim28) .
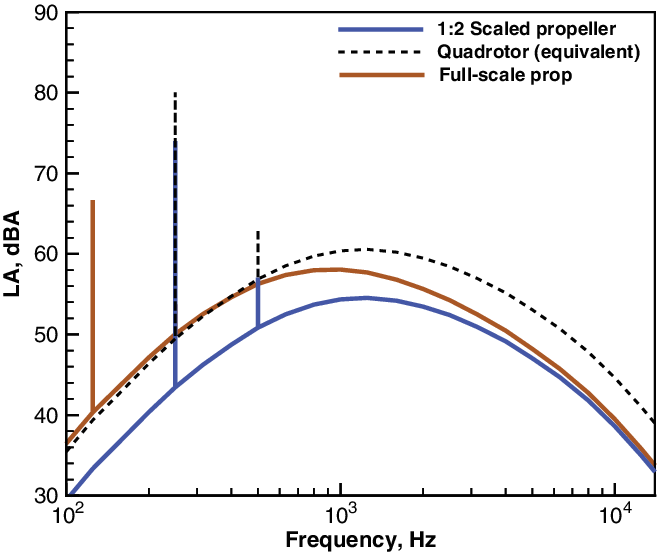
Figure 2. Acoustic emission of a single propeller versus quad-rotor. Full-scale propeller: diameter
$=$
1.96m, blades
$=$
6, rpm
$=$
1,200; axial speed
$V =$
10m/s at
$z =$
100m; receiver at 45deg, with source-receiver distance
$=$
142m. Single propeller trimmed to 900kW; scaled propeller trimmed to 225kW; the quad-rotor delivers 4
$\times$
225kW at rpm
$=$
2,400. No atmospheric attenuation accounted for.
The scaled propeller is a simple 1:2 geometric scaling, but the tip speed is maintained constant, e.g. the rpm is doubled. When the rpm is doubled, we see a shift in tonal components toward higher frequencies, due to the increased blade passing frequency, BPF
$= n \,\mbox{rpm}/60$
. Thus, LA
$_{\rm max}$
increases, and the corresponding frequency is higher. Note that in this case the overall diameter of the rotor system is increased by at least 1/0.65
$=$
1.53, assuming there is no rotor overlap.
The result in Fig. 2 refers to a simple acoustic analysis, which may bear little relation on how this difference in noise may be perceived. Research is progressing with psycho-acoustics aspects(Reference Christian29), to identify how spectral contributions different from aircraft noise will affect annoyance, and hence acceptance by the general public(Reference Fidell, Mestre, Schomer, Berry, Gjestland, Vallet and Reid30). Tonal noise dominates at frequencies
$f <$
1kHz, but tones are moved toward higher frequencies, up to 2.5kHz, because of the high angular speed of the propellers: The smaller the diameter, the faster the spinning rate, as in the example above, Fig. 2. This is where human ears are more sensitive. With the increasing pressure to reduce the impact of aviation, new vehicles cannot be allowed to operate within an unregulated airspace. One clear issue is that of “proximity” of the air vehicle to people on the ground, not least the “fear of danger”, something that has been long established for conventional aircraft(Reference Fields31). Nevertheless, public perception on noise will change in time and even the quietest of vehicles could be seen as a major source of noise pollution
Many electrically powered vehicles are erroneously branded as “zero emissions”. Battery recharging must rely on an electrical network which is likely to be powered by non-renewable resources. Furthermore, the whole life-cycle of the rare-Earth materials required for high- performance batteries and electrical systems are likely to be as polluting as fossil fuels.
Fallacy #4: Urban air vehicles are safe and inexpensive
No serious accident has yet occurred that involved loss of life (on board or on the ground), though several instances of space invasion have been reported, notably around commercial airfields—and mayhem followed(32). Safety considerations for a variety of vehicle architecture have been addressed in Ref.(Reference Courtin and Hansman33), who correctly address the enhanced risks of multi-rotor eVTOL systems, in contrast with fixed-wing airplanes and helicopters.
There are many instances in the history of aviation when a new technology, or a landmark project were stopped as a consequence of critical failures. There is no proof anywhere that urban air vehicles will be safe, no way of assessing the intrinsic safety of the vehicles, or their safety within the context of urban integration. Any legacy metric use for commercial aviation is not applicable to this new airspace. A recent memo from the UK Civil Aviation Authority(34) provides a safety risk assessment for all unmanned air systems having a weight greater than 20kg, although there is no specific consideration of manned air systems, since these systems are rapidly evolving at the time of writing. There is no past record of human error with eVTOL, and even a small number of incidents created by these new systems could sink the impressive safety records of modern aviation(35).
The integration of state-of-the-art technologies into vehicles that have not been designed and optimised for redundancy is likely to put a stop to the urban air vehicle concept as soon as there is n accident. Currently, there is no certification procedure (airworthiness, operational limitations) in place for any urban air vehicles carrying payload or passengers to prevent air accidents involving eVTOL. The outlook is very different from the early days of aviation, when prototypes were operated from rural areas. By contrast, the urban air vehicles are meant to operate within densely populated areas with high traffic levels.
What are the recovery options in case of mechanical failure? Several prototypes featuring many independent rotors lack the redundancy level typical of multi-engine helicopters, which require shaft power to be delivered via a central shaft powered by all engines. With this architecture, single-engine failures are not so critical; failure modes and recovery strategies for engine failure are not demonstrated anywhere in the technical literature. Furthermore, auto-rotation is not possible for most of the vehicles proposed, which indicates that a hull loss is very likely. The prototypes developed around the world tend to have single or co-axial propellers individually powered by electric motors. These concepts have the advantage of minimising costs by using off-the-shelf components and use distributed electric propulsion.
Fallacy #5: New electric propulsion technologies are ready to be used in aviation
Electric propulsion can be scaled up/down and distributed across several power hubs; it offers benefits in comparison with a single power plant, because the power/weight ratio is essentially constant and not dependent on altitude. Gas turbine engines and internal combustion engines do not scale well with size; in the power range they become totally unfeasible. This scale-independence of electrical motors allows designers to generate conceptual designs across a wider range of weights(Reference Marden and Allen36), to distribute propulsion where it is needed, instead of relying on complex power trains. However, the opposite effect takes place on propellers, which become more efficient when the disk area increases (tip speeds; Reynolds number effects, blade design). This power architecture generates independent loads which are difficult to manage, particularly in the presence of a single-point failure or torque imbalance. Heat transfer is a limiting factor as we try to increase the power density.
Fallacy #6: These new urban air systems are opening new markets
It is unlikely that the air delivery of groceries, books and consumer products will ever be profitable. Only high-value and time-critical payloads are realistic, where their cost is competitive with conventional transport systems. There is the possibility of selling the air delivery services at a loss, provided a gain can be achieved by creating new demand elsewhere in the supply chain. Electronic interference, collisions with infrastructure (buildings, electricity pylons), travel over sensitive areas (airports, industrial complexes, hospitals, schools) are unsolved legal problems(Reference Villasenor37).
Two-man personal vehicle configurations shown in the specialised literature do not make it clear whether one is a qualified pilot, or whether the vehicle is to be controlled by anyone on-board. Pilot skills for such vehicles are completely unexplored, and are certainly no lower than for helicopters. For passenger transportation, it is unlikely that eVTOL will be able to make helicopters obsolete any time soon. Whenever a market exists, helicopters can deliver the services of personal transportation. There remains the problem of affordability; on the other hand it is not yet clear how an eVTOL would be more affordable if aviation regulations and certification processes will be in place for these new vehicles as they are for conventional rotorcraft.
4.0 GENERAL DISCUSSION
The current research on the prospects of electrically powered VTOL vehicles is broadly divided into two categories: the vehicle itself and the infrastructure around, e.g. anything external to the vehicle that is necessary to guarantee flight operations. We discuss critical items in the former category only, which is the main motivator of this contribution. There are already some simulation and optimisation programs that allow the exploration of the design space, as demonstrated for example in Refs(Reference Johnson and Silva12,Reference Vegh, Botero, Clark, Smart and Alonso13,Reference Basset, Vu, Beaumier, Reboul and Ortun38), and sophisticated aero-acoustics codes that allow the prediction of noise footprints(Reference Casalino, van der Velden and Romani39). The published literature demonstrates that simulation tools in search of new applications exist. However, the eVTOL application needs tools, data and multi-disciplinary know-how that do not yet exist.
4.1 Direct operating costs
Vehicle sizes range from small drones (W
$\sim$
1kg) for light services (reconnaissance, air delivery of small parcels), to full scale vehicles capable of passenger transportation, with and without pilots (W
$\sim 10^3$
kg). For the sake of discussion, we will refer to the heavier vehicles, which is where major technology challenges are. These vehicles are proposed as air-taxis, available on demand for a ride-share from anywhere to anywhere. Rides are potentially available for a single passenger. Let us make an assessment of potential costs, referring back to the Über example, where a helicopter can be hired on demand, like a taxi(5). A modern utility helicopter would cost $1,500–$2,000 per flight hour. Assuming a short ride of
$\sim$
12 miles with a cruise speed of
$\sim$
80kt, the ride would take about 12–15 minutes, accounting for the all phases of flight, including rotor engagement and rotor stop. Assume another 5 minutes, at best, for passenger embarkation and disembarkation, and the rotorcraft can operate three such flight within the hour. Thus, one ride would cost 500–670 dollars—not exactly a fare that many would be prepared to accept. For an affordable, reliable and dependable air-taxi service, we would need to propose much lower costs with reliability at least on a par with conventional rotorcraft, and environmental emissions markedly lower. Yet, a number of recent presentations in high-profile conferences address sequencing and scheduling of eVTOL aircraft(Reference Yang and Wei40,Reference Bertram, Yang, Brittain and Wei41) , on the basis that a high level of on-demand traffic will soon materialise and would magically help solve congestion issues on the ground.
As far as passenger transport is concerned, a rotorcraft vehicle would have very limited capability; as things stand, Fig. 1, the unit capacity is limited and can only be increased by numbers of aircraft in the air, which would lead to an explosion in air traffic. For example, the EHang 184 eVTOL prototype, designed to transport a single passenger with a
$\sim$
100kg weight, does not require a pilot. The vehicle would start by pressing a button, and then it would flown by a mission control centre, with the passenger not having any control whatsover. This scenario completely neglects a vast array of problems that have not been solved, the most obvious being the cost of transporting this single passenger: How is the the mission control centre accounted for?
By contrast, research on distributed-propulsion fixed-wing (for example the NASA X57 or Joby S2) could be a short-range vehicle capable of transporting 8–12 passengers over 50–100 nautical miles, and thus feed into a hub for onward travel. High utisisation rates are necessary to make even these vehicles viable(Reference Moore and Fredericks42), but this is no different from a Boeing B737 or an Airbus A320.
4.2 Propulsion system: Propellers
Most aviation propellers and all helicopter rotors operate at constant rpm, and have variable pitch control. There are rather complex, as they require an electronic control system and extensive software testing to undergo certification. Electrically powered VTOL using distributed propulsion concepts are likely to operate with variable rpm and fixed pitch, but are less costly, have fewer moving parts and are believed to be subject to fewer faults. An interesting comparison is shown by Duffy et al(Reference Duffy, Wakayama, Hupp, Lacy and Stauffer21) between a hypothetical Boeing eVTOL and the rotor hub of the Robinson R44 (a two-bladed teetering rotor), but the performance of the eVTOL would be limited.
The relationship between power and thrust is different between the two propellers. Fixed-pitch propellers have poor performance in skewed flight, since they have no articulation (flapping, pitching). Lack of articulation causes stronger blade stall on the retreating blade and unbalanced load among blades, which leads to an intrinsic rolling moment. A solution often seen to overcome roll moment is by using counter-rotating propellers. It is noted that this is not the best aero-mechanic solution, because it merely cancels an unwanted effects with another unwanted effect (blade stall).
Even in axial flight there are some interesting issues. A notional propeller having the performance envelope shown in Fig. 3 indicates that moving from the optimum point A (highest efficiency at
$\theta = 30^{\circ}$
) along a line of constant pitch angle (point B) leads to a reduction of propulsive efficiency (
$\eta \simeq 0.70$
), as the output power increases and the advance ratio decreases (as a result of an increase in rpm). By contrast, a variable-pitch propeller can maintain efficiency over wider range of power outputs. For example, it can deliver the same power at the same advance ratio with an efficiency
$\eta \simeq 0.80$
, point D; or it can deliver power at the same advance ratio, and the same speed with two different operation points, C and E, both having higher efficiency than constant-pitch point B. Therefore, the aerodynamic advantages of fixed-pitch propellers are dubious. As a first step, either two-step propellers will be used with cruise and take-off/landing settings, or novel blade configurations that self-regulate their pitch.

Figure 3. Propeller performance map: diameter
$=$
0.98m, blades
$=$
6, design rpm
$=$
2,400.
4.3 Propulsion system: Distributed propulsion efficiency
The efficiency losses of a fixed-pitch propeller, Fig. 3, must be seen in the wider context of the integrated system. A conventional propulsion made of propeller, gear-box and gas turbine engine would have an overall efficiency
$\eta\sim 0.25 \div 0.30$
, at best (efficiencies are: propeller
$ = $
0.82
$\div$
0.85; gear-box
$= 0.94 \div 0.96$
; gas turbine engine
$ = 0.3\div 0.35$
). With distributed propulsion, an efficiency double this value could be achieved,
$\eta = 0.6 \div 0.65$
(propeller
$ = 0.67\div 0.70$
; electric motor
$ = 0.95 \div 0.97$
, gear-box
$ = 0.95$
).
Thus, there are efficiency gains from a correctly integrated distributed electrical system, although this bears no relation as to whether such a vehicle is commercially viable.
4.4 Aero-mechanics
eVTOL come into two broad categories: those that are purely vertical lift vehicles (lifted by rotors only), and those that feature some wings, and are thus able to mix lifting capabilities of both rotors and dynamic lift, depending on the flight regime. In hover, only direct lift is possible; in fast level speed, the wings should be able to provide all the required lift. In between these two extremes, the aircraft must be able to change configuration by tilting the wings. The Rolls-Royce eVTOL falls into the latter category, whilst the Airbus eVTOL (CityAirbus) falls in the former category (multi-rotor electrically-driven helicopter). However, there are also hybrid designs named slowed-rotor winged compound helicopters, which feature both fixed-wing and helicopter rotor, such as the Piasecky PA-890(Reference Quackenbush, Wachspress, Moretti, Barwey, Lewis and Brentner43). Updates are available at the Vertical Flight Society website.6
A number of other configurations seen as prototypes use flight conversion as a key operational capability. This means that the aircraft must be capable of safe transition from rotorcraft to aircraft mode, and back, with sufficient excess power, without stalling or sinking, due to ground effects, gyroscopic effects or pitching moment divergence. This handling quality is not easily guaranteed.
An interesting review of prior art is proposed by Karem Aviation(Reference Karem and Waide44), with some commercial interests on the use of tilt-rotor configurations for eVTOL. None of the above-mentioned problems is noticed. An example is shown in Fig. 4, which is a comparison between an aero-mechanic simulation model for a tilt rotor aircraft (NASA XV-15) and experimental data. These results are rather contrived, in that they are derived using steady-state aerodynamic and flight mechanics analysis. In practice, the speed of conversion (rate of change of the shaft angle) is essential.

Figure 4. Conversion corridor of a tilt-rotor from vertical take-off to level flight; the squares are experimental data(Reference Appleton, Filippone and Bojdo45).
Determination of experimental data at any scale would require time to become available, and there is the possibility that they are very much dependent of the rotorcraft architecture—hence they cannot be extrapolated to other configurations, and the numerical methods cannot be tested.
It is contended here that the design of convertible rotors is not yet a mature science, in spite of decades of effort, and there is no clear certification process available. This situation is not going to change with the use of electrical power.
4.5 Scaling issues
At the very small scale (as low as insect-size), there is limited success with the development of prototypes capable of hovering and performing simple functions; these systems rely on flapping or rotary wing systems, with the latter proving successful down to 0.1kg (Prox Dynamics PD100 helicopter used for military applications). They are more likely to deliver information via sensors operating in critical environments than payload capability; they are designed to rely heavily on autonomy(Reference Maharbiz and Sato46,Reference Wood47) . At a higher weight scale, there are commercial drones, conventional quad-rotor configurations with a full-up weight
$\sim$
10kg, delivering parcels, not without mishaps. Instead, the research focus must be on air vehicles with mass of the order of 100
$\div$
1,000kg, for which there is no mature technology. This is a size between commercial drones and full-scale helicopters: these are visible, pervasive, useful but potentially dangerous vehicles. It is not always clear that there is a technology gap between a drone-size vehicle that can be purchased commercially and a full size vehicle navigating over complex terrain with passengers on board.
A general utility helicopter powered by a turboshaft engine has a specific power of
$\sim$
0.2kW/kg; some brushless electric DC motors can provide at least one order of magnitude higher power output—having a power supply on board. Thus, the use of electrical propulsion, where feasible, opens up new opportunities, but only if we carefully account for the power source, e.g. the battery.
4.6 Autonomy or piloted vehicles
There has been debate for over 20 years about taking physical persons out of the flight platform and how to fly these vehicles out of sight of a ground-based operator (unmanned systems). At the very small scale, this is inevitable. At the commercial scale, an initial consensus has emerged, which proposes a number of stages of accommodation into an evolving air space.
At present, there are no manned or unmanned electric VTOL vehicles that have been certified or undergone testing with data that are open source, but progress is being made.
It concerns the writers that autonomous systems are proposed for personal air vehicles—the flavour of the moment that has replaced the flying car concept that was popular until recently(Reference Moore48).
On a more technical basis, one of the central tenets around urban air vehicles is the question of flight autonomy—not something new, in fact. However, there seems to be unbounded faith in completely autonomous vehicles capable of navigating through complex terrain to fulfill their mission(Reference Floreano and Wood49).
What level of autonomy is strictly necessary? This question appears not to have been answered, but classifications have been proposed (sensor, reactive and cognitive autonomy) and considerable work already exists. In view of the integration with the urban network, urban air vehicles could be programmed to operate exclusively on a fixed trajectory between two points. But if the flight platform is to navigate above sparsely populated areas, then more flexibility can be gained with autonomous systems. The issue with autonomy is that the flight system must be able to learn to operate in a specific environment. Machine learning inevitably requires analysis of a considerable amounts of data, some deterministic and others not; if there is no history, the learning process will require a very long time. At the present time, we must not forget how difficult is to certify self-driving road vehicles. More specifically, in the field of aviation, the activity that has been central to vehicle certification has been extensive flight testing on a deterministic basis. Using large scale probabilistic analysis is something completely new that will take time to develop and accept at a regulatory level.
Let us focus more closely on the certification. Who is the relevant authority? In the current geo-politics, it is unlikely that there will be worldwide agreement, especially if communications will be based on the next generation wireless data networks. There could be a European, a Chinese and an American standard, not necessarily compliant with each other. With more and more demonstrations of formation flight carried out by drones, and with the use of data-mining and self-learning algorithms, one can foresee the growth of autonomous vehicles and the reduction of piloted vehicles. Immense amounts of data will be generated, the ownership of which has not been clarified.
4.7 Environmental issues
Some eVTOL are defined as near-silent. How silent? There is no way of knowing, since there are no published noise prediction methods for this class of aircraft, and no measured data in the public domain. The first-order analysis shown in Fig. 2 indicates that the acoustic effects are not negligible.
There is very low public acceptance of noise generated by helicopters and aircraft flying over built-up areas. Some informal recommendations point to a 15dB noise reduction compared with vehicles of comparable weight. Currently, there is no acoustic compliance requirement for drone-size vehicles. The density, position, number and speed of the number of vehicles present over an area, need to be considered. The noise of helicopter saving a life is more tolerable than the noise of several vehicles flying for recreation, or transportation.
Visual Threat: Would the reader be confident that one such a vehicle flying in close proximity is not a menace, if not an outright threat? How much new air traffic is the public prepared to bear? This is not just a question of flight planning, aerial warfare(Reference Dunn50) or cyber security analysis(Reference Javaid, Sun, Devabhaktuni and Alam51). The number of scenarios leading to incidents is far wider than the civil aviation has witnessed, and aviation case laws have already indicated a number of unintentional consequences(Reference Bellows52). Society has still to form an informed opinion on the acceptance and risks(Reference Clothier, Greer and Metha53), which is understandable, since we are yet to witness the development of these new vehicles, but there is the distinct possibility of an explosion in new air laws to respond to litigation and enforcement(Reference Hodgkinson and Johnson54).
There are unanswered social and psychological questions, privacy and security concerns. In an increasingly busy world of 24-hour operations, expanding this new air traffic into the night will create immeasurable problems, and eventually litigation against the industry.
Energy Use: The use of electrical power is branded as zero-emissions technology, with the automotive industry leading the way. On the contrary, battery charging requires energy from somewhere else, which is likely to be supplied via source that may or may not be renewable. The production, servicing and disposal of batteries requires the use of energy and high-value materials and chemical compounds, which may or may not be sustainable(Reference André and Hajek55). Most often there is no proper accounting of the environmental credentials of these new systems. Airworthy electric components and systems are becoming an important strand of research.
5.0 CONCLUSIONS
Widespread optimism permeates the debate on the future of aviation, where electrically powered rotorcraft systems are concerned. Everyone wants to get to market ahead of everyone else with minimum risk and cost; therefore, there is presently considerable commercial interest. The interest of established airframe manufacturers alongside newcomers to the field is healthy and enriches the aviation eco-system with new talent.
Some white papers call this new age Aviation 4.0, or the age of democratisation, although the democratisation of flight came about in the 1990s with the no-frills airlines that opened up routes to minor airports at extremely low prices. The true cost of the new eVTOL is still unknown.
The research published so far appears to lean on one side only: either the development of the air vehicle, or the development of the infrastructure. The advocates of either strand of research often neglect external factors in the complex aviation environment.
In this paper, we have demonstrated that this optimism relies on several fallacies. Some clever mathematics has been demonstrated to work out a plethora of problems surrounding design, power availability, operations, logistics and economics, but the results are often misleading. There are projections of moving from ground traffic to “smart urban air-mobility”, as if in the sky there will not be traffic and no need for regulations. Strict flight regulations covering eVTOL operations must be in place as a matter of urgency. To avoid excessive costs, the regulatory framework cannot grow in an organic way as it happened with the road networks over long periods of time.
The air transport of passengers will always be expensive in comparison with other modes of transport, and it will not alleviate congestion within large metropolitan areas. If congestion relief is to be addressed, that will be done with mass transport systems on the ground. Speed is welcome, but not at all costs. The market for personal air transportation is limited, the real costs are hidden to the potential customers, the certification issues are often set aside, a large number of engineering problems remain unsolved, and a comparison with modern helicopters is neglected in order to justify the use of electrical power for brand new VTOL aircraft.
In the authors’ view, the demise of the helicopter as a personal urban vehicle is not forthcoming any time soon; this rotorcraft has a demonstrated safety record in the transport of passengers, both civil and military.
However, there exist possibilities of harnessing novel technologies such as distributed electrical power, alongside conceptual design, to develop new vehicle systems that could complement the services offered by conventional rotorcraft. These vehicles are likely to be smaller and lighter than current VTOL, and thus could offer new services at a competitive cost, not necessarily the transport of passengers. These services are likely to include airborne reconnaissance of building sites, off-shore installations, infrastructure that is difficult and expensive to reach, border surveillance, delivery of high-value time-sensitive payloads with high-frequency services, delivery across water and difficult terrain, last-mile delivery where landing options are not available to full-scale aircraft, weather monitoring, along with a vast array of military operations that is not necessary to describe. The best way forward is to first identify application areas that can benefit from new VTOL aircraft and then pursue personal air mobility. Although the eVTOL have enormous unexplored possibilities and a massive design space, it is likely that some lessons will have to be learned by developing and testing some of the new technologies on conventional helicopters. Detailed simulation studies and tests of actual hardware could complement this effort.
Since the barrier-to-entry to the aerospace industry has been lowered by the possibility of developing sub-scale flight systems, there are many new players, not all with the knowledge required to drive the technology forward, or with a clear vision of what can be delivered, what are the safety implications and the certification requirements. Many important problems are discounted by discipline specialists as trivial or obvious. The history of aviation demonstrates that Obvious is the father of all disasters.
ACKNOWLEDGEMENTS
This work has been generated from the UK Vertical Lift Network, EPSRC Grant EP/M018164/1, and the project Methods and Experiments for Rotorcraft (Mentor), EPSRC Grant EP/S010092/1.