Lidar high-density survey and measurement (HDSM; Opitz and Limp Reference Opitz and Limp2015) in archaeology tends to operate on two scales: (1) a small geographic scale, measured in square meters, focused on a single feature or site with data collected by handheld, mobile, or tripod-mounted terrestrial lidar; and (2) a large geographic scale, measured in square kilometers—often with thousands of sites—with data collected using airborne lidar mounted on a piloted aircraft. Between these two scales is a medium geographic scale, measured in hectares, rather than square meters or kilometers. Until recently, this scale was problematic because high-resolution terrestrial lidar is impractical for anything except small areas, but archaeological interpretations based on lower resolution airborne lidar are limited.
A platform that works well at a medium geographic scale is lidar acquired by unpiloted aerial vehicles (UAV). UAV lidar is currently rare in archaeology (Barbour et al. Reference Barbour, Sassaman, Almeyda Zambrano, Broadbent, Wilkinson and Kanaski2019; Opitz and Herrmann Reference Opitz and Herrmann2018; Poirier et al. Reference Poirier, Baleux and Calastrenc2020; Risbøl and Gustavsen Reference Risbøl and Gustavsen2018; VanValkenburgh et al. Reference VanValkenburgh, Cushman, Castillo Butters, Rojas Vega, Roberts, Kepler and Kellner2020), but it is on track to being much more common as it becomes less expensive (see Casana et al. [Reference Casana, Laugier, Hill, Reese, Ferwerda, McCoy and Ladefoged2021] for details on how to build and launch lower-cost UAV lidar for archaeology). What does this technology offer archaeology? What can we do with UAV lidar that we cannot already do with lidar data from other platforms? And, how do we capitalize on lidar data from small, medium, and large geographic scales?
Our aim is to begin to answer these questions by presenting a method for using UAV-acquired lidar in conjunction with lidar data collected by other platforms and scales to study monumental architecture. The size of construction projects has long been used as a cross-cultural proxy for degrees of social control (Trigger Reference Trigger1990) and the materialization of ideology (DeMarrais et al. Reference DeMarrais, Castillo and Earle1996). This is based on the premise that monuments, palaces, and religious architecture—unlike other large structures such as fortifications for the common defense of a settlement—represent the capacity of societal factions to organize and control labor, often for purposes that benefited the elite. In the Hawaiian Islands, it is exceedingly rare that the apparent volume of building material is reported, despite the fact that there are hundreds of temples (heiau) and other large structures distributed across the archipelago. This is understandable given how difficult it is to assess volume in the absence of detailed topographic survey and excavations. Without size data, however, we are limited in what the history of monument building can tell us about societal transformations.
MAKAHIKI AND STATE RELIGION IN THE HAWAIIAN ISLANDS
Makahiki, as it was practiced at European contact in AD 1779, was an integral element of Hawaiian state religion. It was an annual religious season when war was suspended, and elites would make a circuit of the island carrying a symbol of the god Lono to collect tribute in the form of surplus food and fine goods (Figure 1). The largest temple at Kealakekua, Hikiau Heiau, was dedicated to the war god for most of the year, but during the Makahiki season, it was used by the “cult of Lono”—a special sect of priests associated with fertility, agriculture, and peace—who would open and close the season on the heiau (temple; Malo Reference Malo1951). These ceremonies would have been opportunities for elites to display their grandeur and redistribute collected tribute beyond their normal cadre of retainers. The British accounts from AD 1779 describe their hosts providing them with an abundance of food, goods, and hospitality at Kealakekua.
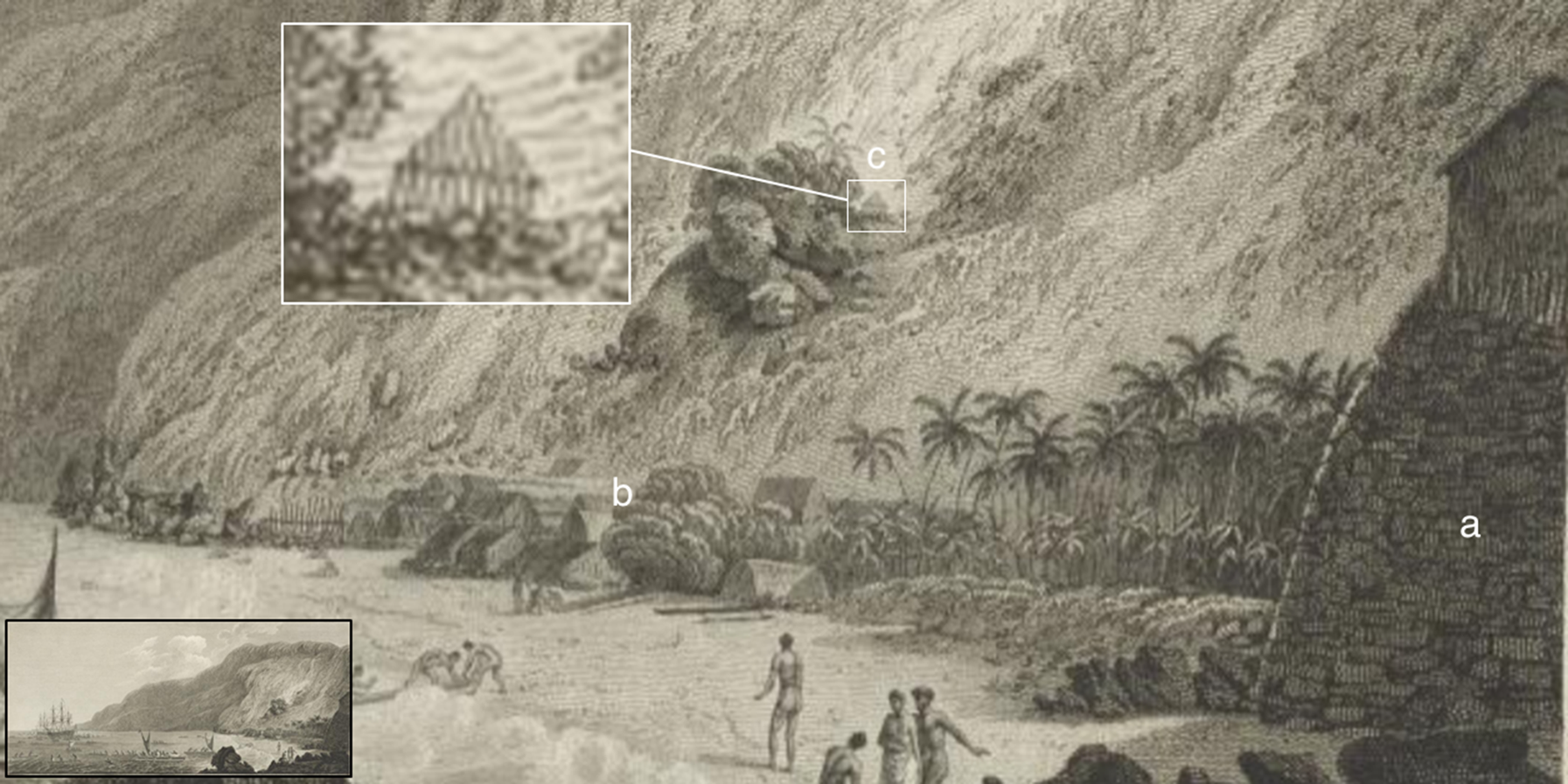
FIGURE 1. Drawing of the Royal Center at Kealakekua in AD 1779. This image shows (a) a temple—Hikiau Heiau—and (b) houses of priests. Although most of the enclosing wall around the religious precinct is not visible from the viewer's perspective, (c) a structure situated on a terrace on the slopes of Nāpo‘opo‘o Valley may be the North Section of the Great Wall. (Source: “A View of Karakakooa in Owhyee” by John Webber [Reference Webber1784]. National Library of Australia.)
Although there is good evidence that Makahiki was practiced across the Hawaiian archipelago, there are few direct accounts of it outside of Kealakekua that can be linked to specific cultural sites (McCoy Reference McCoy2018). Historical linguistics suggest that the Makahiki religious tradition evolved from rituals practiced by ancestral Polynesians (Kirch and Green Reference Kirch and Green2001:57–60) millennia before the first permanent settlers arrived in the Hawaiian Islands around AD 1000 (Kirch Reference Kirch2011). But neither linguistic reconstructions nor oral history tells us when it took on the form described by Europeans and the first generation of Hawaiian historians employing the written medium. We are, therefore, without a strong material basis for determining when the Makahiki festival became “[institutionalized] as a means of tribute collection by the emerging archaic state hierarchy” (Kirch et al. Reference Kirch, Mertz-Kraus and Sharp2015). Most scholars suspect that it occurred late in the precontact era, around AD 1550–1700—a time when we see a spate of temple construction that, along with other evidence, suggests that society was undergoing a shift from a chiefdom to an archaic state with a divine monarch acting as the head of a state religion (Hommon Reference Hommon2013; Kirch Reference Kirch2010).
A key archaeological metric for understanding the history of Makahiki is the massive wall that enclosed the religious precinct at Kealakekua. It defined a 3.3 ha area used for residences for priests, a sacred pond, the massive Hikiau Heiau, and the smaller Helehelekalani Heiau (for a summary, see Hommon Reference Hommon2014). Archaeologists working in the Hawaiian Islands refer to walls like this one as a “great wall.” There is no formal definition of a “great wall.” It is simply used to distinguish traditionally built walls—usually more than several meters thick and several meters high—from other large walls, such as stone walls built to control livestock in the postcontact era. The Kealakekua Great Wall has appeared on maps for more than a century, but there are conflicting accounts of the true size of the feature (Figure 2). In addition, it is covered in thick vegetation, it has been modified to varying degrees by later land use, and previous studies have suggested that some portion of the wall may be buried by erosional deposition. Excavations have been extremely rare in the religious precinct, and prior to our research, there were no reported reliable radiocarbon dates associated with any architecture from this—or any—of Hawaii's royal centers (for a recent review, see Reith et al. Reference Reith, Hunt, Lipo and Wilmshurst2011).

FIGURE 2. Previous maps of the Royal Center at Kealakekua. The footprints of Hikiau Heiau and the three-sided Great Wall around the religious precinct are shown. In this study, we derived a more accurate and precise rendering of both structures using terrestrial and airborne lidar.
In many ways, the Great Wall at Kealakekua is like other examples of monumental architecture in the Hawaiian Islands and around the world: there are conflicting accounts of its size, little detail recorded on its form, and no secure date for its construction. Here, we describe how we used UAV-acquired lidar, along with terrestrial and piloted lidar platforms, to map the Great Wall at Kealakekua. Lidar allowed us to estimate the amount of building material used in its construction, compare it with other large structures—including nearby Hikiau Heiau—and visualize its form. Carefully placed excavations proved important for both ground truthing building-rubble estimates and collecting material to radiocarbon date to determine the likely date of the wall's construction. In this case, lidar proved its utility beyond just detecting architecture through thick vegetation by (1) giving us a way to estimate building material regardless of the uneven preservation of the architecture and (2) helping detect and quantify building material buried by erosional deposition.
MONUMENTAL ARCHITECTURE IN THE ROYAL CENTERS OF KONA DISTRICT, HAWAI'I ISLAND
There are few well-dated early cultural deposits from the Kona District on Hawai'i Island. Paleoenvironmental reconstructions based on pond cores at Hōnaunau suggest “major growth in settlement and activities” along the coast around AD 1450 (Athens et al. Reference Athens, Ward and Blinn2007:iii). Salvage excavations have yielded six radiocarbon dates on short-lived taxa, with the earliest estimated to represent activities around AD 1600–1830 (cal AD 1521–1815; 230±40 BP, Beta-279222; Reith Reference Reith2011). Unfortunately, these dates are not associated with any architecture, and none of the previous efforts at dating monument construction at Hōnaunau (Ladd Reference Ladd and Pearson1969a) meet current chronometric standards (for a recent review, see Reith and Athens Reference Reith and Athens2013).
On the basis of genealogical estimates, the first king who united Hawai'i Island, ‘Umi-a-Līloa, came to power around AD 1570–1590 (Kirch Reference Kirch2010). He broke with tradition and moved the island's political center from the windward valley of Waipi‘o to the leeward coast of Kona (Kamakau Reference Kamakau1961:34; Kirch Reference Kirch2010:168). Over the next two centuries, between AD 1600 and the arrival of Captain Cook at Kealakekua Bay in AD 1779, six royal centers were built along a 30 km stretch of the Kona coast at Kailua, Kahalu‘u, Keauhou, Hōlualoa, Kealakekua, and Hōnaunau (Cordy Reference Cordy2000). It is unclear if Kealakekua was the center of Makahiki ceremonies prior to the capital's shift to Kona, or if it became the center some time afterward.
Kona's royal centers were partially documented by Bishop Museum ethnographer John Stokes in 1906, who recorded more temples in Kona (n = 59) than any other district on the island. Detailed mapping by Stokes (Reference Stokes1991) and by Henry Kekahuna in the 1950s provides an especially valuable source of information about religious and royal centers prior to large-scale development and reconstruction (see also Cordy Reference Cordy2000; Hommon Reference Hommon2013, Reference Hommon2014). In the 1960s and 1970s, a resurgence of interest in royal centers led to a number of surveys (e.g., Emory Reference Emory1986; Hommon Reference Hommon1969; Soehren and Newman Reference Soehren and Newman1968) and excavations (Ladd Reference Ladd and Pearson1969a, Reference Ladd and Pearson1969b; Soehren and Tuohy Reference Soehren and Tuohy1987) carried out by the Bishop Museum on behalf of the National Park Service and Hawai'i State Parks.
Previous archaeological surveys in Kona's six royal centers suggest that although each had a unique layout and special features, there were some common elements (Stokes Reference Stokes1991). These centers were occupied by elites and included royal compounds (or “palaces”; e.g., Flannery Reference Flannery, Feinman and Marcus1998), temples (heiau), sledding tracks (holua), compounds for priests, grounds for training and sports, fishponds, and mausoleums, all surrounded by a dense population to support the center. Centers also included massive dry-masonry stone “great walls” to delineate important areas.
At the sacred refuge (pu‘uhonua) of Hōnaunau, some 5 km south of Kealakekua, the surrounding “great wall” easily falls within the largest size category of traditional Hawaiian architecture (Figure 3). The wall has a reconstructed length of 305 m, and it is on average 5.2 m wide and 3.7 m tall, with an apparent volume of 19.25 m3 per linear meter (LM) of wall (Kirch Reference Kirch1985). The apparent volume is, however, somewhat larger than the actual volume of building material because the wall's architects used the “pao technique,” creating voids within thick sections of the wall to save on fill. Nonetheless, the entire wall's apparent volume, 5,864 m3 (estimated from reconstructed length × average profile height and width), makes it one of largest structures ever built in the independent kingdom of Hawai'i Island. The war temple Pu‘ukoholā Heiau, for example, has an apparent volume determined by terrestrial lidar of 5,023 m3 (Mulrooney et al. Reference Mulrooney, Ladefoged, Gibb and McCurdy2005).
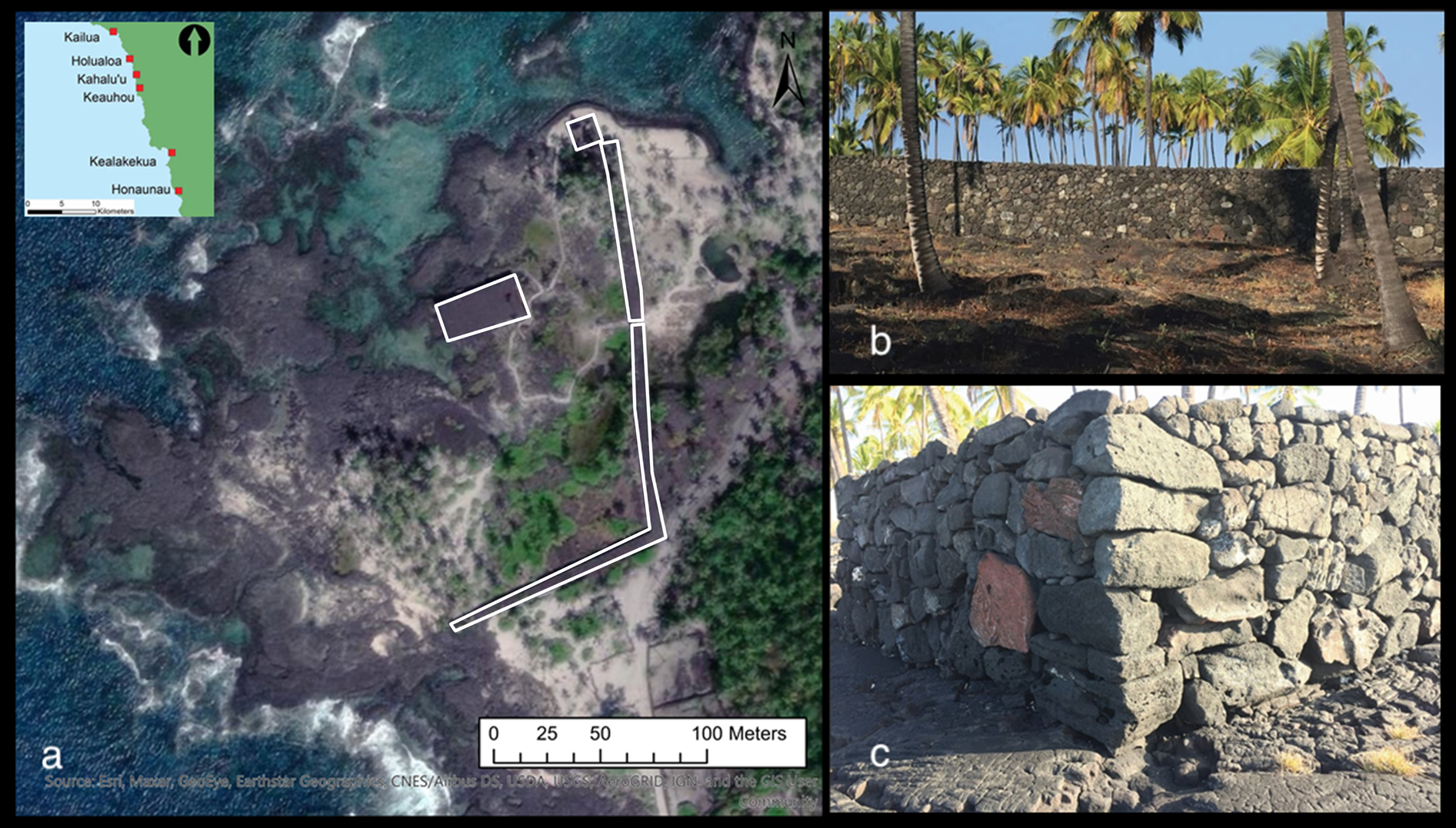
FIGURE 3. The Great Wall of the Royal Center at Hōnaunau, Kona District, Hawai‘i Island. This reconstructed two-sided wall is the best-known example of a “great wall” in the Hawaiian Islands. It was used to enclose a sacred refuge with a major temple at the center and a royal mausoleum at the northern end of its East Section. There is a formal entry slightly off from the center of the wall's East Section, and as at Kealakekua, the enclosed area is open on the coastal side. (Photos [b, c] by Mark D. McCoy.)
Other Hawaiian kingdoms had even larger structures. On Maui, there were at least five temples with larger volume estimates (Kolb Reference Kolb1991, Reference Kolb1994, Reference Kolb1997, Reference Kolb1999), including the largest freestanding stone architecture built in the archipelago—Pi‘ilanihale Heiau, at 21,937 m3. Other large temples include Popoiwi Heiau (11,098 m3), Halekai‘i Heiau (9,515 m3), Pihana Heiau (6,686 m3), and Loaloa Heiau, which was estimated by Kolb (Reference Kolb1991) as 5,834 m3 and by Kirch and colleagues (Reference Kirch, Holson and Baer2010) as 10,400 m3. But, due to a lack of systematic study, it is difficult to contextualize these volume estimates in terms of local architectural traditions (i.e., form, style) or the broader cross-cultural pattern of monument size and power (e.g., Trigger Reference Trigger1990).
SITE DESCRIPTION
Located in Kealakekua Bay, Nāpo‘opo‘o is a small coastal valley with a steep talus slope along its northern and eastern edges. The mouth of the valley was home to a royal center with a large religious precinct. Today, the area is managed by the State Parks of Hawai'i in collaboration with the local nonprofit group Ho‘ala Kealakekua. Regular maintenance activities involve clearing vegetation, but in the past, there were several major efforts to restore Hikiau Heiau. The wall around the religious precinct has not been the target of reconstruction, and today it remains obscured under thick vegetation. In addition to the architecture that remains from the precontact era, many of the stone walls in Nāpo‘opo‘o Valley likely date to the historic era, and they were built to control livestock and mark property boundaries.
There are conflicting accounts of the form and size of the wall around the religious precinct at Kealakekua (Figure 2). In 1906, Stokes (Reference Stokes1991) mapped a total of 340 m of wall in two segments—a North Section about 164.5 m long and an East Section about 175.5 m in length with a structure near its center. An earlier 1883 map, however, appears to show a 130 m long South Section extending to the ocean, but it fails to show the other sections of the wall. Beginning in the 1960s, archaeological maps show all three sections (Soehren and Newman Reference Soehren and Newman1968). Due to many years of development along the road leading to Kealakekua Bay, however, the South Section now falls short of reaching the coastline.
The first detailed map of all three sides of the wall show segments of widely varying sizes (Yent Reference Yent1985: Figure 25). The estimated profile of the East Section was reported to be as much as 5 m wide and 2.5 m high. In the North Section, the freestanding wall changes form to become a terrace at the base of a talus slope. The portions of the South Section that are still visible were described as a low and poorly preserved freestanding wall. In addition to the poor state of some sections of wall, there is good reason to suspect that some of the feature may be buried by erosional deposition. Hommon (Reference Hommon2014:46), citing excavations in the area by Yent (Reference Yent2006), notes “the depth of the alluvial deposits suggests that the great wall may have served not only to delineate the boundary of the sacred Hikiau precinct but also to protect it from flooding and slopewash.” When we began our research, however, there had never been any excavations targeting the wall itself.
METHODS
We begin with a description of lidar acquired by piloted airborne lidar, unpiloted aerial vehicle (UAV) lidar, and tripod-mounted terrestrial lidar (Table 1). Next, we summarize excavations around the Great Wall at Kealakekua aimed at collecting datable material in good archaeological context and determining how much building material may be below the present ground surface. A full report on the details of the excavation can found in McCoy and Ladefoged (Reference McCoy and Ladefoged2018). Finally, we briefly describe two of the techniques used to calculate volume estimates: image mensuration and building height (ArcGIS Pro, ESRI). We describe elsewhere how to build and fly UAV lidar for archaeological survey, and we discuss in detail the data processing workflow (Casana et al. Reference Casana, Laugier, Hill, Reese, Ferwerda, McCoy and Ladefoged2021).
TABLE 1. Summary of Survey Lidar Platforms: Terrestrial, Unpiloted Airborne, and Piloted Airborne.

Note: This table represents datasets relevant for mapping the Great Wall at Kealakekua. Each survey examined other areas as well.
a Point densities are postprocessed ground points only.
b Inertial measurement unit.
Lidar Datasets
Lidar data came from three sources: piloted airborne lidar, unpiloted aerial vehicle (UAV) lidar, and tripod-mounted terrestrial lidar (Figures 4 and 5). The piloted lidar was originally flown for the U.S. Federal Emergency Management Agency (FEMA) to create a baseline dataset for coastal flooding management for the entire island. The UAV lidar results are from 10.56 ha across the Nāpo‘opo‘o Valley in Kealakekua Bay. Terrestrial lidar scans were conducted on Hikiau Heiau and on a well-intact portion of the East Section of the Great Wall.
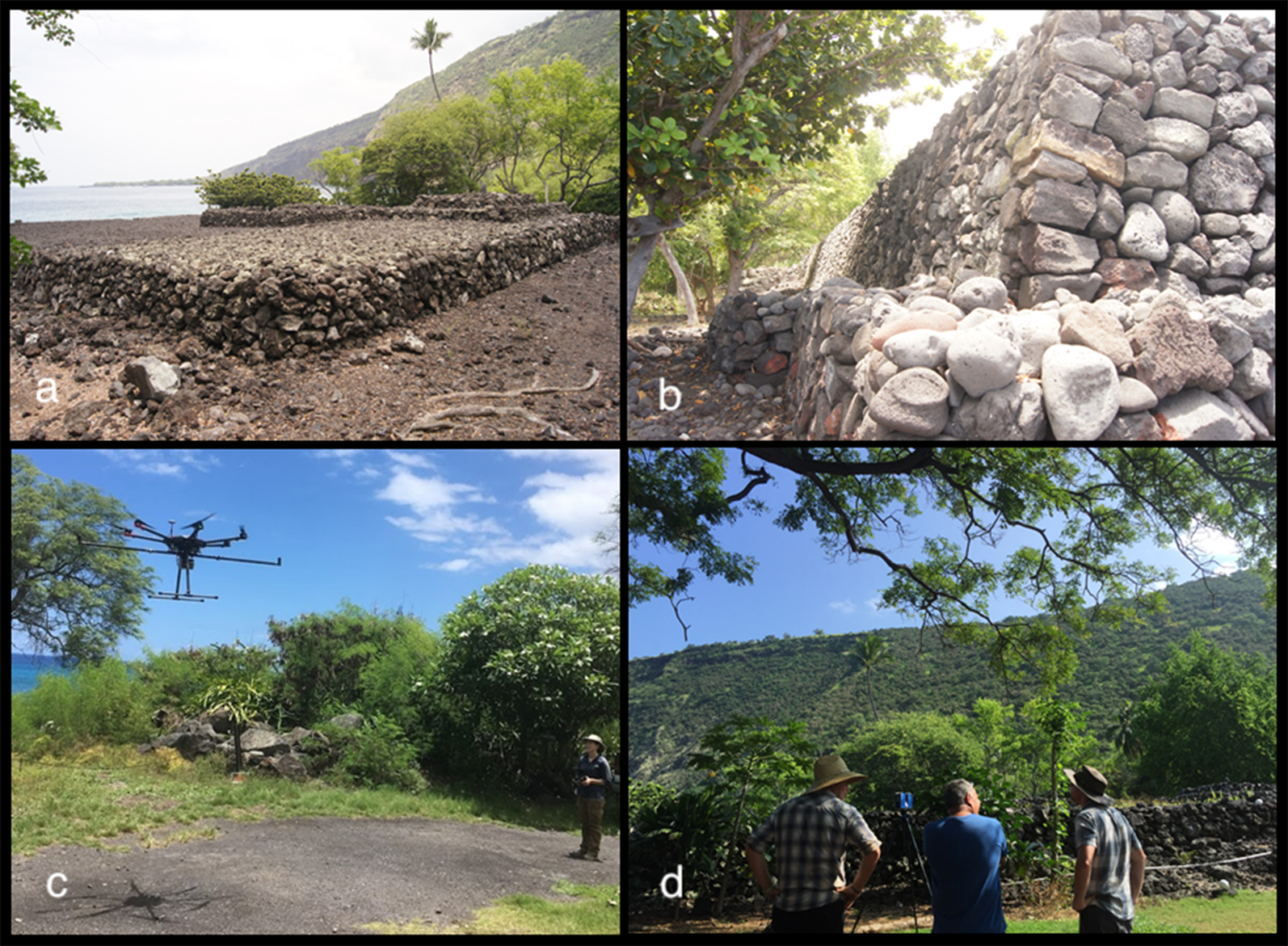
FIGURE 4. Lidar survey in Kealakekua, Hawai‘i Island. Hikiau Heiau is large temple build on a natural outcrop. Much less building material was required on the (a) southeast corner compared with the (b) northwest corner. Survey using lidar mounted on a (c) UAV and (d) tripod were used to estimate the volume of building material for Hikiau Heiau and the Great Wall. (Photos by Mark D. McCoy.)

FIGURE 5. Types of lidar survey data. For the study area, we have (a) lidar data from a piloted coastal survey by FEMA (1 point per m2) that covers Kealakekua Bay, (b) UAV lidar (30 points per m2) for Nāpo‘opo‘o Valley, and tripod-mounted terrestrial lidar (for this study, decimated to 1,058 points per m2) for Hikau Heiau and a section of the enclosing wall (outlines of surveyed areas shown).
Piloted Airborne Lidar
In 2006, a lidar survey was flown by Airborne 1 Corporation (El Segundo, California) along the coast of Hawai'i Island up to about 200 m above sea level. The target point spacing was 1 m. Ground control points were used for GPS-derived orthometric height (GEOID12A). A quality check of the dataset was published along with the bare-earth and raw point clouds. The 2006 FEMA Lidar: Hawaiian Islands Point Cloud files with Orthometric Vertical Datum GPS-derived orthometric height via GEOID model using GEOID12A is available for download from NOAA's Office for Coastal Management (https://coast.noaa.gov/dataviewer/#/).
Terrestrial Lidar
In 2016, we conducted two weeks of field survey and terrestrial lidar scans of architecture in Kealakekua, including Hikiau Heiau (Figure 6) and a section of the Great Wall (Figure 7; McCoy Reference McCoy2017a). Footprints of architecture were recorded by GPS (Trimble GeoXH GPS) and control points were taken to later georectify previous survey maps. Prior to terrestrial scanning, each area of interest had to be cleared of vegetation by hand in combination with chain saws and leaf blowers. A Faro Focus was set at stations spread across each study area, and 6-inch (15.25 cm) spheres were used as controls to merge 50 station scans. Complete models (.ply) were exported from Faro's proprietary software and imported into the open-source software Cloud Compare (version 2.11). Models were further cleaned, converted to meshes, and decimated to 1,058 points per m2. Apparent volume was estimated using the Measure Volume function in Cloud Compare (tool is located in Edit > Mesh > Measure Volume).

FIGURE 6. 3D model of Hikiau Heiau. Inset is the altar on the east end of the temple.

FIGURE 7. 3D model of East Section of Great Wall, Kealakekua. A small segment of intact wall facing is visible. Note that the current ground surface is consistently about 60 cm higher on the upslope eastern side of the wall.
Unpiloted Aerial Vehicle Lidar
In 2019, our team flew a UAV lidar survey over Nāpo‘opo‘o, Kealakekua (McCoy et al. Reference McCoy, Ladefoged and Casana2020). This survey utilized a Geodetics Geo-MMS lidar system carried on a DJI M600 drone. The Geo-MMS system utilized a Velodyne VLP-16 sensor integrated with a proprietary IMU and two dual-frequency GNSS receivers. One 20-minute flight was undertaken at an altitude of 40 m above ground level with a transect spacing of 50 m. The drone traveled at 2 m per second and collected an average of 561 points per m2. Flight planning and autonomous mission control was accomplished with UgCS Pro. Raw data processing to integrate Post-Processed Kinematic (PPK) GNSS data and generate .las point cloud output was accomplished with the Geodetics LidarTool software. Processing the resulting point cloud to generate a bare-earth Digital Terrain Model (DTM) was accomplished through a combination of SAGA GIS and LAStools. The resulting point cloud data covered 10.56 ha (0.11 km2) and 30 ground points per m2. Elevation above sea level was corrected using bare-earth airborne lidar flown by FEMA in 2006 (UTM, NAD 1983, Zone 4N. Vertical Datum: NAVD88, Vertical Units: Meters).
Excavations
In 2018, we conducted small test excavations to determine when the Great Wall at Kealakekua was constructed. Our goal was to recover cultural deposits that predate the enclosing wall's construction (i.e., deposits from under the wall) and deposits that represent a period when the wall was in use. In brief, we recovered cultural deposits typical of habitation activities under the East Section that we interpreted as representing earlier settlements. On the North Section, natural outcrops at the base of cliffs were used to create stone- and earth-filled terraces, including a stone-filled terrace with distinctive depressions that we interpret as having held wooden carvings (ki‘i) of substantial sizes. We excavated cultural deposits from on top of an earth- and stone-filled terrace on the North Section that likely represent activities dating to when the wall was in use. We had charcoal screened for short-lived species—a necessary step for eliminating long-lived taxa—and report here the first reliable radiocarbon dates from the royal center at Kealakekua.
Apparent Volume Estimates for Architecture
We experimented with several different techniques to estimate the apparent volume of architecture—both intact stone and displaced rubble—using our terrestrial lidar scan of Hikiau Heiau as a quality control. We began by determining the volume of the Hikiau Heiau model in Cloud Compare (4,234 m3). Next, it was exported to ArcGIS Pro using the Import 3D Files function (.obj to multipatch) then saved as a TIF to test the image mensuration function (see topic “Image mensuration” in ArcGIS Pro). The ArcGIS Pro image mensuration tool produced a volume estimate for the terrestrial lidar scan identical to the Cloud Compare mesh volume estimate (4,188.45 m3 [cut] + 45.27 m3 [fill] = 4,233.72 m3 total volume; Figure 8).

FIGURE 8. Techniques for estimating apparent volume for architecture: (a) image mensuration and (b) building height. Image mensuration technique gives a total volume (cut + fill) for a selected area. Building height technique requires creating a buffer of elevation points and then creating an estimated ground surface below the feature to estimate the height of material. In the case of Hikiau Heiau, there is a clear contrast between the southeast, where little building material was required (~1 m building height), and the northwest (~4 m building height).
We also estimated volume using a method for deriving building height from lidar (see topic “Obtaining elevation information for building footprints” in ArcGIS Pro). It required first mapping the footprints of architecture. In this case, we created an outline of Hikiau Heiau and the Great Wall based on a hillshade of the UAV bare-earth digital elevation model (35 cm resolution). Next, a buffer (polygon) around the footprint was created representing the zone greater than 1 m but less than 2 m from features. The UAV-lidar-based DEM was converted to a point layer, and the buffer was used to select a ring of points around each feature. These elevation points were used to create an interpolated (IDW method) estimate of the ground surface under the feature. In the final step, the difference between the estimated ground surface and the DEM gave positive and negative height values that were converted to apparent volume (cut and fill) in a point layer.
The building height technique described here allows for high-resolution visualizations of features as well as volume estimates (Figure 8). For example, if a single point had a height of 2.5 m, given that it represented a 0.35 × 0.35 m area, the volume at that point is 0.31 m3 (0.35 × 0.35 × 2.5 m). To extend this to an entire feature, one simply selects all the points and sums them. To extend the example above, if a feature had 10 points with that same height, its total volume is 3.1 m3.
RESULTS
Although the building height technique for estimating volume produces a good visualization of the amount of building material—as opposed to image mensuration, which only produces a quantity metric—it did not produce a good volume estimate for Hikiau Heiau. The building height technique total volume estimate for Hikiau Heiau was 11.7% lower than that determined by image mensuration of terrestrial lidar (3,726.8 m3 [cut] + 11.8 [fill] = 3,738.6 m3 total volume). In contrast, when the image mensuration tool was applied to the UAV lidar of for Hikiau Heiau, it produced a result that was within 3% of the terrestrial lidar volume estimate (4,276.7 m3 [cut] + 50.7 m3 [fill] = 4,327.4 m3 total volume).
Our UAV lidar data image mensuration estimate for the extent and volume of the Great Wall at Kealakekua suggests that it was the largest structure on Hawai'i Island at the time of European contact. Our total estimate, in which we compensate for buried sections of wall and rubble as well as impacts of modern development, puts the apparent volume at 6,147 m3 (Figure 9). This is based on an average of 15.7 m3 per LM of wall and a total length of 391.5 m. This includes 342.5 m of freestanding wall (East Section: 167.5 m; South Section: 175 m) that would have been on average 5 m wide and 3.14 m tall, with an additional 49 m in the North Section where the wall becomes a terrace along the lower slopes of the valley. This estimate presumes that the South Section would have extended to the coastline and that the basalt outcrop that defines the coastline in this location has been stable.

FIGURE 9. Estimated building height for the Great Wall at Kealakekua. The current wall is, on average, just over 2 m tall, but our results suggest that it had an average reconstructed profile of 3.14 m high × 5 m wide × 391.5 m long, with an apparent volume at 6,147 m3.
Although UAV-acquired lidar was the primary instrument we used to arrive at our volume estimate, terrestrial and piloted airborne lidar were also important. The terrestrial lidar scan of a 30 m portion of the East Section yielded an image mensuration average volume of 13.7 m3 per LM of wall and a maximum height above the ground surface of 3.25 m. The scans also suggested that the upslope side of the wall may have had a great deal of accumulated erosion given that the modern ground surface was slightly, but consistently, much higher than the downslope side. Excavations on the upslope side of the wall confirmed that there were at least 62 cm of accumulated soil and stone (Figure 10). We recovered a nineteenth-century metal nail fragment under wall fall next to foundation stones, which suggests that this rubble began accumulating after the abandonment of the religious precinct. For our image mensuration volume estimate, this meant that we needed to account for not only several courses of buried foundation stones—about 1.6 m3 per LM based on a width of 5 m—but also about 0.4 m3 per LM of wall-fall stones that had accumulated next to the wall and been buried by erosional deposition. This is how we arrived at an average of 15.7 m3 per LM of wall (13.7 m3 above ground; 2.0 m3 below ground).

FIGURE 10. Excavations of the Great Wall at Kealakekua. Test Unit #1 on the upslope side of the wall showed at least two courses of buried foundation stones and a great deal of rubble (Layer I-B) and slope wash (Layer I-A) that had accumulated after the feature had been abandoned. Below the rubble, we uncovered a thick cultural deposit (Layer II) that extends under the wall. The cultural layer, which was also found in shovel tests more than 20 m upslope of the wall, likely represents activity prior to the wall's construction. In this profile, we distinguish between the portion of the cultural deposit that had eroded from upslope of the wall and accumulated at the foot of the wall (Layer II-A) and the undisturbed deposit that runs completely under the wall (Layer II-B). Test Unit #2, on the opposite side of the wall, did not have slope wash, and the cultural layer was recorded as Layer II.
Our UAV airborne lidar allowed us to determine independently if there is enough rubble today to justify the average volume determined by terrestrial lidar and excavations. The image mensuration technique was applied to all sections of the wall within the UAV lidar flights (227.5 m of freestanding wall and 30 m of terrace). The apparent volume 11.0 m3 per LM of wall found (2,422.1 m3 [cut] + 409.3 m3 [fill] = total volume 2,831.4 m3) can be thought of as a “minimum” volume estimate because it does not account for buried wall, rubble, or robbed stone. Compared with our estimate, this result is an underestimate of 2.7 m3 per LM (visible above ground), or about 20% less material than one would expect based on the best-preserved portion of wall. The building height technique also gave a volume slightly less than expected, with an average of 12.1 m3 per LM (2,867.2 m3 [cut] + 254.3 m3 [fill] = total volume: 3,121.5 m3). We have not attempted to determine a “maximum” volume estimate because that would be best accomplished through further excavations and careful consideration of the original dimensions of each segment of wall.
Although airborne lidar image mensuration estimates are lower than what we would expect, they nonetheless confirm that our average of 15.7 m3 per LM of wall is reasonable. This assumes that up to 20% of the original volume of stone is currently somewhere outside of the estimated extent of rubble due to both natural degradation and the robbing of stone to create other walls. We note that there are hundreds of meters of walls, likely built during the historic era, within the valley.
To determine the likely date of construction, we radiocarbon dated material from cultural deposits under the wall as well as deposits on top of the wall (Figure 11). The excavation on top of a terrace on the North Section yielded a calibrated date of AD 1640–1800 with a narrow intercept at cal AD 1646–1669 (2σ; Table 2). Historic records give us independent evidence that the wall was in use by AD 1779 (terminus ante quem). The new radiocarbon results indicate that deposits began accumulating on top of the wall no earlier than AD 1640 (terminus post quem). The cultural layer below the wall's foundation stones included materials consistent with precontact habitation in this location (i.e., charcoal, faunal bone fragments, and volcanic glass and basalt flakes). Four dates on charcoal from short-lived plants from this layer yielded ranges from cal AD 1440 to 1640 (2σ); earliest intercept at cal AD 1446–1514 (Table 2). This fits with the expectation that, as at Hōnaunau, there was a coastal settlement around AD 1450, a date that coincides with dates on coral offerings in upland gardens beginning in AD 1441 ± 19 (AGEG-2016-55; McCoy et al. Reference McCoy, Mulrooney, Horrocks, Cheng and Ladefoged2017). The coincidence of the end of the accumulation of habitation deposits below the East Section of the wall and the beginning of dates from deposits on the North Section of the wall point to a construction date of around AD 1640.

FIGURE 11. Estimated date of the construction of the Great Wall: AD 1640. Historic records give us a terminus ante quem (TAQ) for the Great Wall of AD 1779. A radiocarbon date from the North Section (light gray) gives us a terminus post quem (TPQ) of AD 1640 because this is the earliest that deposits could have begun accumulating on top of the wall. No dates from under the East Section (dark gray) postdate AD 1640. Therefore, we believe that the TPQ is close to the date of construction.
TABLE 2. Radiocarbon Dating Results, Kealakekua.

Sources: OxCal v.4.3.2 (Bronk Ramsey Reference Bronk Ramsey2017), IntCal13 (Reimer et al. Reference Reimer, Bard, Bayliss, Warren Beck, Blackwell, Ramsey, Buck, Cheng, Lawrence Edwards, Friedrich, Grootes, Guilderson, Haflidason, Hajdas, Hatté, Hoffmann, Heaton, Hogg, Hughen, Felix Kaiser, Kromer, Manning, Niu, Reimer, Richards, Marian Scott, Southon, Staff, Turney and van der Plicht2013).
Note: Dates reported by Waikato Radiocarbon Dating Laboratory (Wk).
DISCUSSION
The availability of lidar by more—and more affordable—platforms means that future archaeological survey will undoubtedly involve datasets collected at a range of resolutions and geographic scales. Today, most lidar studies center on small geographic scale survey (i.e., millimeter resolution data over hundreds of square meters made possible with handheld and terrestrial lidar) or large geographic scale survey (i.e., sub-meter-scale resolution over square kilometers by piloted lidar). UAV-acquired lidar data fills the gap between these two extremes.
One of the things that sets a UAV-acquired lidar survey apart from data gathered using other lidar techniques is the capacity for data visualization. Here, we make the distinction between representative visualization, as in the lifelike images possible with terrestrial lidar, and data visualization aimed at “the discovery of new information, patterns, or relationships among variables through exploratory analyses of spatial representations of data” (McCoy and Ladefoged Reference McCoy and Ladefoged2009:265). Although piloted airborne lidar has been used to map traditional architecture (Ladefoged et al. Reference Ladefoged, McCoy, Asner, Kirch, Puleston, Chadwick and Vitousek2011; McCoy et al. Reference McCoy, Asner and Graves2011), and terrestrial lidar has been used to estimate volume (Mulrooney et al. Reference Mulrooney, Ladefoged, Gibb and McCurdy2005), this study is the first use of UAV lidar in the Hawaiian Islands. The data visualizations possible with 30 points per m2 via the building height technique, but also more commonly used techniques (i.e., Relief Visualization Toolkit; Kokalj and Somrak Reference Kokalj and Somrak2019; Zakšek et al. Reference Zakšek, Oštir and Kokalj2011), provided much more precise for imaging of archaeological features compared with previous airborne lidar datasets.
Twenty years ago, the capacity for piloted airborne lidar to mitigate the problem of vegetation obscuring our view of the landscape kicked off the development of a number of innovative methods to bring out the subtle topography of archaeological features. Many of these efforts have focused on detecting and extracting different types of features from bare-earth models (e.g., Casana Reference Casana2014; Forest et al. Reference Forest, Costa, Combey, Dorison and Pereira2020; Howey et al. Reference Howey, Sullivan, Tallant, Kopple and Palace2016; Kokalj et al. Reference Kokalj, Zakšek and Ošti2011; McCoy et al. Reference McCoy, Asner and Graves2011; Sevara et al. Reference Sevara, Pregesbauer, Doneus, Verhoeven and Trinks2016; Quintus et al. Reference Quintus, Day and Smith2017). These new data, plus the tools to visualize them, changed the way we “see” the archaeological record (Huggett Reference Huggett2020; McCoy Reference McCoy2020a, Reference McCoy2020b; VanValkenburgh and Dufton Reference VanValkenburgh and Dufton2020). In the next stages of the geospatial revolution in archaeology, we will need to find more ways to leverage our steady stream of different kinds of lidar data (McCoy Reference McCoy2017b), create more kinds of visualizations that are useful for archaeology, and carefully consider which remote-sensing techniques are appropriate for the goal of the project (Casana Reference Casana2020).
None of these technologies come with tools to bridge the gap between data collection and the creation of information relevant to answering archaeological questions (for a discussion of data, information, and evidence in the context of geospatial technology, see McCoy Reference McCoy2020a:17–44). In this case, lidar data from other platforms improved and expanded what we could do with the UAV-acquired lidar. Piloted lidar was key to correcting the absolute elevations of the UAV lidar (i.e., meters above sea level) because it had greater geographic range and quality checks against established benchmarks. Terrestrial lidar also proved invaluable as a check against our UAV-lidar-based estimates of the volume of features. Other projects planning to use and evaluate UAV lidar in the future would do well to ground-truth measurements using terrestrial lidar. We would also note that although our focus is on lidar data, there is no reason why photogrammetric models could not also be used as a cross-check on volume estimates.
We are not the first to derive apparent volume for archaeological features. Many of the advances in this aspect of mapping have focused on techniques that are customized to the type of landform and shape of the archaeological target, but they are also limited to methods that are scalable to thousands of structures (Chase Reference Chase2017; Ebert et al. Reference Ebert, Hoggarth and Awe2016; Menze and Ur Reference Menze and Ur2012; Stanton et al. Reference Stanton, Ardren, Barth, Fernandez-Diaz, Rohrer, Meyer, Miller, Magnoni and Pérez2020). In this study, the main target of our survey was one large structure—the Great Wall at Kealakekua. It is unevenly preserved. Some sections are in good condition, whereas others have been extensively modified, robbed of stone, and degraded to rubble. The combination of the geographic scale and resolution of UAV lidar allowed us to not only map even poorly preserved sections but also estimate apparent volume of building material. Lidar, of course, cannot penetrate the ground surface. But terrestrial lidar showed how a small portion of wall had been partially buried by slope wash—a finding that we confirmed through excavation. In sum, although the ArcGIS Pro image mensuration technique gave a reliable total volume (cut + fill) for a selected area, it would require modification to be useful for projects involving thousands of structures.
Our results suggest that the Great Wall at Kealakekua was likely the largest structure built on Hawai'i Island prior to European contact. The feature is 45% larger, by apparent volume, than Hikiau Heiau—which is located within the religious precinct at Kealakekua—22% larger than the war temple Pu‘ukohalā Heiau, and slightly larger than the Great Wall at Hōnaunau. Its design is also notable. It is, for example, the same apparent volume per LM of wall as sections of Hadrian's Wall built in AD 122 to protect the boundary of the Roman Empire in Northern England. But the height of Hadrian's Wall was, in places, greater than its width (i.e., 5 m high × 3 m wide), whereas the Great Wall at Kealakekua is the reverse (i.e., 3 m high × 5 m wide). It is possible that Hawaiian architects tasked with building such a massive structure were aware of the potential hazard that earthshaking would have posed a narrow dry-masonry stone wall. Future studies of monumental architecture within a seismically active area such as the Hawaiian Islands hold a great deal of potential to expand the study of archaeoseismology, a field in which ancient architectural traditions have been used as proxy evidence for the awareness of earthquakes as a natural hazard in the era before seismic instrumentation and record keeping (e.g., Sintubin Reference Sintubin2011).
As a material expression of the power of state religion in Hawai'i, and more specifically the efforts made in service of the Makahiki festival, the Great Wall at Kealakekua is an excellent metric. The limitations of radiocarbon dating mean that we do not know how long it took to build, but we conclude that it was completed around AD 1640. Without high-quality dates in association with architecture from the more than two dozen other royal centers across the Hawaiian Islands, it is difficult to say how Kealakekua fits within the transition to a state society. But, given that oral histories put the shift of the island's capital to the Kona coast slightly earlier, at AD 1600, it may be that Makahiki ceremonies took the form observed at the time of European contact after society was well on its way to transitioning to an archaic state.
CONCLUSION
Lidar for small geographic scale survey using a range of ground-based platforms (handheld, mobile, and tripod-based terrestrial lidar) and large geographic scale survey using airborne platforms (piloted aircraft) are increasingly common in archaeology. We present a method for studying monumental architecture on a medium geographic scale that leverages new UAV-acquired airborne lidar covering more than 10 ha (30 ground points per m2), along with small-scale tripod-based terrestrial lidar and large-scale piloted airborne lidar. We tested a tool for estimating volume (image mensuration, ArcGIS Pro) and adapted a technique for estimating building height from lidar to visualize construction fill. Our targets were a well-maintained temple—Hikiau Heiau—and a massive but poorly documented wall that surrounded the religious precinct within which the temple was situated. UAV-acquired lidar survey data, when paired with excavations and radiocarbon dating, suggests that the Great Wall at Kealakekua was likely the largest structure built on the island prior to European contact in AD 1779. We speculate that the construction of the wall around AD 1640 marks a key point in the evolution of the Makahiki ceremony when it came to resemble how it was practiced at contact, and it suggests that expressions of religious authority continued to evolve during the transition from a chiefdom to an archaic state society.
Acknowledgments
This research was conducted in collaboration with Ho‘ala Kealakekua and supported by a grant from the University of Auckland, along with in-kind support from the Hawai'i State Parks and the Bishop Museum. Special thanks to Tracy Tam-Sing and Gordon Leslie for their kōkua. Mahalo to everyone who lent a hand in making this project a success: Hayley Glover, Rob Hommon, Ann Horsburgh, Jen Huebert, David Ingleman, Adam Johnson, Ben Jones, Patrick Kirch, Gordon Leslie, Keone Leslie, Tania Leslie, Bronson Leslie, Reagan Leslie, Shane Akoni Nelsen, Sarina Pearson, Tracy Tam-Sing, Ben Teele, Charmaine Wong, Martha Yent, and Rose Young. Thanks to Karolyn Buhring and Conny Pellegrino for their help in translating the abstract to Spanish. This manuscript was improved thanks to comments from several anonymous reviewers.
Data Availability Statement
Piloted lidar data is available from NOAA's Office for Coastal Management (https://coast.noaa.gov/dataviewer/#/). UAV lidar and terrestrial lidar data are on file with the State Parks of Hawai'i. Unedited terrestrial lidar is also accessible at Sketchfab for the Great Wall (https://skfb.ly/6UXGr) and Hikiau Heiau (https://skfb.ly/6YSFw). Supplemental UAV-lidar data files are also available at https://figshare.com/s/2a54d841416f7648a72f and https://figshare.com/s/36d0bc2d1fb465fc8ef4.