Summations
-
Neurochemical neurotransmission via habenula may provide clues for developing biomarkers for the DDC function.
-
Selective agonists’ modulation of the secretion of biochemical biomarkers may reflect changes in habenular neurotransmission activity.
-
Most promising biomarkers are linked to nicotine receptor, dopamine D4 receptor, μ-opioid receptor and also to habenular glia cells.
Considerations
-
The polysynaptic trajectory including the DDC has not sufficiently been studied.
-
There is still a lack of concrete research data which biochemical biomarkers can be explored as an indicator of the DDC function.
-
The interaction between the immune system and DDC function is not yet sufficiently crystallised.
Introduction
Attempts to describe how the forebrain regulates certain mental functions often fail due to their almost unbridled complexity. Within the brain, virtually everything seems to be connected to everything else in various directions, and that makes it difficult to disentangle the most important pathways. This is all the more relevant when it comes to the description of the role of concrete cerebral structures in psychiatric disorders in which a variable system of mental functions is affected (Lebow & Chen, Reference Lebow and Chen2016; Hu et al., Reference Hu, Cui and Yang2020; Koob, Reference Koob2021). Apart from general overviews of the results of a large number of scientific studies, these articles usually yield very few useful insights. This is related to a lack of structure to order and value the various findings.
We have argued several times in the past for the use of a model of how the forebrain is organised in the search for the mechanisms that play a role in the development of mental disorders (Loonen & Ivanova, Reference Loonen and Ivanova2018a, Reference Loonen and Ivanova2018b, Reference Loonen and Ivanova2019a). The model we propose to use is derived from the evolutionary history of the different forebrain parts. It is assumed here that the original building plan at a higher system level can provide the structure along which the individual research findings can be interpreted. We assume that structures which arose later in evolution offered the advantage of better tailoring the response to the environmental circumstances, but that it is less likely that they took over regulation according to a completely different approach.
We have yet another starting point, namely that most mental disorders according to the current classification systems do not each have a separate and unique pathophysiological background (Loonen et al., Reference Loonen, Ochi, Geers, Simutkin, Bokhan, Touw, Wilffert, Kornetov and Ivanova2021). In mood disorders, for example, various biological factors can put and keep a person in a depressed state. When a biological or psychological treatment sufficiently weakens the influence of these biological factors, natural resilience mechanisms have a chance to lead to clinical recovery (Stassen et al., Reference Stassen, Angst, Hell, Scharfetter and Szegedi2007). Activation of this resilience mechanism can also occur spontaneously (during placebo treatment). Since antidepressant treatments with completely different targets are all about equally effective in the treatment of Major Depressive Disorder (MDD, Stassen et al., Reference Stassen, Angst, Hell, Scharfetter and Szegedi2007), it appears that the influence of the depressive factor can be attenuated by various pharmacological routes. With the necessary adaptions, this can also apply to various other (neuro)psychiatric syndromes: this makes it desirable to be able to identify those patients where the relevant target point has an optimal impact on psychological functioning. Biomarkers for processes of circuits involving habenuloid complex may well be suitable for this.
In this article, we will first outline the content and background of our model for forebrain functioning. Then we want to apply the evolutionary relationships to get a better understanding of the connectivity with and of the habenuloid complex as described in the scientific literature. The biochemical entities that partly determine the strength of this connectivity may be suitable as biomarkers. In this respect, we will concentrate on examples of factors that influence the process of neurotransmission. The main objective of this paper is to offer clues for future multidisciplinary research.
Model for gross functioning of the forebrain
Evolution of the forebrain in vertebrates
We have reviewed the evolutionary relationships between forebrain structures in detail in earlier work (Loonen & Ivanova, Reference Loonen and Ivanova2015, Reference Loonen and Ivanova2016c). The first vertebrates were jawless animals with a lamprey-like appearance living about 560 million years ago (mya). What is striking when considering the lamprey brain is that already in this early stage an astonishing modern extrapyramidal system existed (Grillner & Robertson, Reference Grillner and Robertson2016) and that the connectivity of the habenuloid complex with the brainstem barely changed during the entire evolution of vertebrates (Batalla et al., Reference Batalla, Homberg, Lipina, Sescousse, Luijten, Ivanova, Schellekens and Loonen2017). The globus pallidus of the lamprey forebrain contains a set of glutamatergic neurons that project on the lateral habenula and are involved in changing behaviour after evaluation of its effects (Stephenson-Jones et al., Reference Stephenson-Jones, Kardamakis, Robertson and Grillner2013). In an amphibian stage of vertebrate development (370 mya), both an amygdaloid and a separate extrapyramidal system existed. The lamprey striatum corresponds to the centromedial amygdala of the frog and the globus pallidus to the bed nucleus of the stria terminalis. This means that the human ‘extended amygdala’ corresponds to the striatopallidum of our earliest vertebrate ancestors. Amphibians do not yet have a cerebral cortex with an input-processing and output-generating role like mammals have. In amphibians, the entire cortex-like pallium is still part of an extensive ‘limbic’ forebrain control system (Roth et al., Reference Roth, Grunwald and Dicke2003; Laberge & Roth, Reference Laberge and Roth2007; Laberge et al., Reference Laberge, Mühlenbrock-Lenter, Dicke and Roth2008). The amphibian extrapyramidal system corresponds to the human ventral extrapyramidal system, including the nucleus accumbens and ventral pallidum. The six-layered cerebral cortex, which occupies a dominant position within the human brain, emerged relatively late during evolution in early mammals (145 mya) (Rakic, Reference Rakic2009). During the mammalian part of evolution, the so-called isocortex has grown massively: in apes, the cerebral cortex takes up almost three quarters of the brain (Van Dongen, Reference Van Dongen, Nieuwenhuys, Ten Donkelaar and Nicholson1998; Voogd et al., Reference Voogd, Nieuwenhuys, Van Dongen, Ten Donkelaar, Nieuwenhuys, Ten Donkelaar and Nicholson1998). In parallel, the dorsal thalamus and dorsal extrapyramidal system (including caudate nucleus and putamen) increased in size enormously (Butler, Reference Butler1994a, Reference Butler1994b; Grillner & Robertson, Reference Grillner and Robertson2016).
The habenula and its connections to the interpeduncular nucleus in the brainstem are of even an earlier date than the first vertebrates. They are described in the hagfish (Jansen, Reference Jansen1930; Wicht & Nieuwenhuys, Reference Wicht, Nieuwenhuys, Nieuwenhuys, Ten Donkelaar and Nicholson1998) which, evolutionarily, is an ancestor of the lamprey (Loonen & Ivanova, Reference Loonen and Ivanova2015). Although these data are relatively old and therefore not entirely reliable, presumably the ‘ganglion habenulae’ of the hagfish involves mainly the medial cholinergic part of the habenula in later vertebrates. In the lamprey, there is clearly a lateral division that indirectly affects dopaminergic and serotonergic nuclei in the midbrain (Stephenson-Jones et al., Reference Stephenson-Jones, Floros, Robertson and Grillner2012). From this initial beginning of the vertebrates, this pathway through which the forebrain influences the activity of ascending monoaminergic neurons from the midbrain retained its importance.
Considering these relationships, we propose to distinguish a primary, secondary and tertiary forebrain. The primary forebrain essentially consists of the hippocampal complex, the amygdaloid complex (corticoid and extended amygdala), the hypothalamus and the habenuloid complex and more or less represents the lamprey-like forebrain. The primary forebrain initiates the essential behaviours which are crucial to continue life of every individual and of our species. These behaviours are related to nourishing, defending and reproducing in the broadest sense. Salience attribution is, for example, an important function of the primary forebrain. The secondary forebrain consists of specific parts of the medial prefrontal cortex, the corresponding cortico-striato-thalamic-cortical circuits which include the core and shell parts of nucleus accumbens and determine the ventral extrapyramidal system, limbic parts of the dorsal thalamus as well as again the habenuloid complex (Fig. 1). The secondary forebrain motivates the individual and regulates the readiness to the behaviours initiated by the primary forebrain. Appetitive reward-seeking and distress-avoiding fleeing behaviours are, for example, functions of this part of the forebrain. The primary and secondary systems are intricately connected, for example: the hippocampus projects heavily on the shell part of the nucleus accumbens (Heilbronner et al., Reference Heilbronner, Rodriguez-Romaguera, Quirk, Groenewegen and Haber2016), while this shell part projects, in turn, to the extended amygdala (Heimer et al., Reference Heimer, Zahm, Churchill, Kalivas and Wohltmann1991). The tertiary forebrain consists of the remainder of the cerebral cortex, the dorsal cortico-striato-thalamic-cortical circuits, the remainder of the dorsal thalamus and to a certain extent the habenuloid complex. This part of the forebrain interprets sensory input and initiates specific cognitive and/or behavioural output which serves to adapt to the ever-changing circumstances within the individuals’ biotope. Memorisation and training as well as thought and reasoning are an integral part of these processes. Again, this tertiary part is connected with the majority of structures of the primary and secondary forebrain and can adapt their functioning which would lead to the advantage of more sophisticated regulation of their functions depending on the specific circumstances.
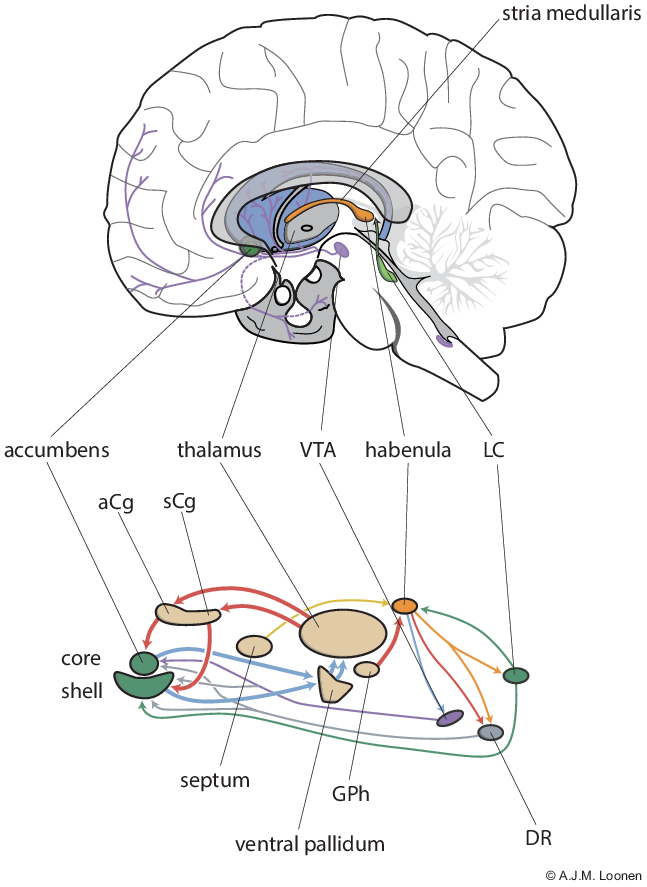
Fig. 1. Circuits regulating pleasure and happiness. The diagram below depicts the ventral extrapyramidal cortico-striato-thalamo-cortical circuitry that controls the willingness and intensity of reward-seeking and distress-avoiding behaviour. Also shown are the structures that influence these circuits. The figure above shows the position of some components in the human brain. aCg = anterior cingulate cortex; DR = dorsal raphe nucleus; GPh = human equivalent of habenula-projecting globus pallidus; LC = locus coeruleus; sCg = subgenual cingulate cortex; VTA = ventral tegmental area.
The habenula is not a trivial part of either the primary or secondary forebrain, since it can be considered to represent the essential part of the dorsal diencephalic conduction (DDC) system between forebrain and upper brainstem. Within the tertiary forebrain, direct connectivity via the medial forebrain bundle between the prefrontal cortex and particularly dopaminergic neurons of the upper brainstem, represents the ventral conduction system (Geisler et al., Reference Geisler, Derst, Veh and Zahm2007; Watabe-Uchida et al., Reference Watabe-Uchida, Zhu, Ogawa, Vamanrao and Uchida2012). The habenula is a small, bilaterally paired nuclear complex of the epithalamus; in humans it measures only 20–30 mm3 in each hemisphere. Despite its small size, the habenula is highly complex in composition (Schmidt & Pasterkamp, Reference Schmidt and Pasterkamp2017). In both rats and mice, the habenula consists of a large number of individual nuclei and domains that can be divided into a medial and lateral division (Aizawa et al., Reference Aizawa, Kobayashi, Tanaka, Fukai and Okamoto2012; Wagner et al., Reference Wagner, Stroh and Veh2014). In addition, the habenuloid complex is asymmetrical in size in many animal species and although this is less the case in humans, the existing asymmetry may well still apply to the function in humans (Hétu et al., Reference Hétu, Luo, Saez, D’Ardenne, Lohrenz and Montague2016; Ahumada-Galleguillos et al., Reference Ahumada-Galleguillos, Lemus, Díaz, Osorio-Reich, Härtel and Concha2017). These characteristics suggest that the currently applied research methods are too crude to provide a sufficiently nuanced picture of the role of this complex in the regulation of human behaviours and the creation of (neuro)psychiatric clinical pictures. This is one of the reasons for our plea to look much more carefully at the role in primitive vertebrates such as the lamprey and the frog when studying the role of the habenula in humans (Loonen & Ivanova, Reference Loonen and Ivanova2018b). This can provide useful insights into the importance of seemingly insignificant connectivity, and therefore with associated specific biomarkers and new treatment options.
Connectivity of the habenula
The anatomical, physiological and functional connectivity of the habenula has recently been extensively reviewed (Batalla et al., Reference Batalla, Homberg, Lipina, Sescousse, Luijten, Ivanova, Schellekens and Loonen2017; Zahm & Root, Reference Zahm and Root2017; Fakhoury, Reference Fakhoury2018; Aizawa & Zhu, Reference Aizawa and Zhu2019; Metzger et al., Reference Metzger, Souza, Lima, Bueno, Gonçalves, Sego, Donato and Shammah-Lagnado2021). An overriding problem in anatomical studies is that – due to the multitude of connections – projections from small, but essential, structures can be hidden from view. Moreover, it is mainly neurons of monosynaptic projections that are mapped: it may be much more interesting to study the place of the habenula as central relay in the chain of neurons that constitute forebrain-to-brainstem neuronal pathways. It is therefore important, in our opinion, to take the phylogenetically old organisation as a starting point and to consider whether their course and functions have changed during evolution and how phylogenetically younger structures interact with them. An important point here is that based on its relative age, it can be assumed that the habenula plays a role primarily in modulating the function of the primary and secondary forebrain. Its involvement in the pathogenesis of (neuro)psychiatric disorders is then certainly not inconceivable (Loonen & Ivanova, Reference Loonen and Ivanova2018a).
Having said this, the medial habenula appears to receive input mainly from the bed nucleus of the anterior commissure and triangular nucleus in the posterior septum and gives output to, respectively, the peripheral and central parts of the interpeduncular nucleus (Aizawa & Zhu, Reference Aizawa and Zhu2019). The input from these septal areas is primarily cholinergic and this neurotransmitter stimulates amply expressed nicotinic acetylcholine receptors (Shih et al., Reference Shih, Engle, Oh, Deshpande, Puskar, Lester and Drenan2014; Batalla et al., Reference Batalla, Homberg, Lipina, Sescousse, Luijten, Ivanova, Schellekens and Loonen2017). In humans, the medial habenula is far smaller than the lateral habenula (which accounts for about 95% of the total volume), but still consists of several subnuclei (Aizawa & Zhu, Reference Aizawa and Zhu2019). Purinergic neurotransmission may also be involved as fibres of the triangular septal nucleus release ATP as a fast neurotransmitter (Aizawa & Zhu, Reference Aizawa and Zhu2019). Moreover, μ-opioid receptors are expressed in distinct regions of the medial and lateral habenula and synthesis of interleukin 18 has also been observed (Sugawa et al., Reference Sugama, Cho, Baker, Joh, Lucero and Conti2002; Gardon et al., Reference Gardon, Faget, Chu Sin Chung, Matifas, Massotte and Kieffer2014; Aizawa & Zhu, Reference Aizawa and Zhu2019; Metzger et al., Reference Metzger, Souza, Lima, Bueno, Gonçalves, Sego, Donato and Shammah-Lagnado2021). The majority of the habenular-interpeduncular output is taken care of by cholinergic (ventral MHb) or peptidergic (substance P-ergic, dorsal MHb) neurons (Aizawa & Zhu, Reference Aizawa and Zhu2019) which can both co-release glutamate (Ren et al., Reference Ren, Qin, Hu, Tan, Qiu, Zhao, Feng and Luo2011). Within the interpeduncular nucleus, acetylcholine predominantly affects nicotinic cholinergic receptors and muscarinic M2-class autoreceptors on cholinergic terminals (Batalla et al., Reference Batalla, Homberg, Lipina, Sescousse, Luijten, Ivanova, Schellekens and Loonen2017).
In their detailed review, Zahm & Root (Reference Zahm and Root2017) summarise the results of a large number of (anatomical) studies on the afferent and efferent connectivity of the lateral habenula and relate these as much as possible to their association with subnuclei. The majority of the input to the lateral habenula comes from a continuum of nuclei extending from the lateral preoptic to the lateral hypothalamic region and probably including the ventral pallidum and bed nucleus of the stria terminalis as well (Zahm & Root, Reference Zahm and Root2017). The output from the lateral habenula goes mainly to the rostromedial tegmental nucleus (RMTg), various monoaminergic centres and to the laterodorsal tegmental and incertus nuclei. The GABAergic RMTg has an important inhibitory effect on dopaminergic projections from the midbrain, but also within the ventral tegmental area (VTA) itself habenular efferents predominantly activate inhibitory neurons (Li et al., Reference Li, Fan, Liu, Shen, Liu and Zhao2021). In addition, moderate LHb input comes from the mesial prefrontal cortex (extending from anterior Cingulate cortex (aCg) via subgenual Cingulate cortex (sCg) to the anterior insular cortex), the entopeduncular nucleus (globus pallidus interna in primates) and the upper part of the brain stem (Zahm & Root, Reference Zahm and Root2017). There is still uncertainty about the significance of projections from the lateral septum; these could be connections between the hippocampal complex and the lateral habenula as we have suggested before (Batalla et al., Reference Batalla, Homberg, Lipina, Sescousse, Luijten, Ivanova, Schellekens and Loonen2017; Zahm & Root, Reference Zahm and Root2017). The majority of the lateral habenula neurons are glutamatergic, but a small-celled medial core is enriched with neuropeptides (substance P, vasopressin, somatostatin, neurotensin) (Zahm & Root, Reference Zahm and Root2017). In Batalla et al. (Reference Batalla, Homberg, Lipina, Sescousse, Luijten, Ivanova, Schellekens and Loonen2017) and in Loonen and Ivanova (Reference Loonen and Ivanova2018b), a scheme is presented for the chemoarchitecture for this connectivity in which we took the relationships of ancient forebrain as a reference. With certain adaptations (Zahm & Root, Reference Zahm and Root2017; Metzger et al. Reference Metzger, Souza, Lima, Bueno, Gonçalves, Sego, Donato and Shammah-Lagnado2021), this scheme is still valid (Fig. 2).

Fig. 2. Chemoarchitecture of the dorsal diencephalic conduction system. Different neuronal connections use more than one neurotransmitter for the transmission of impulses. This is not indicated in the diagram. The peptidergic output of the IPN has not been adequately assessed. (Adapted from Batalla et al., Reference Batalla, Homberg, Lipina, Sescousse, Luijten, Ivanova, Schellekens and Loonen2017, Loonen & Ivanova, Reference Loonen and Ivanova2018a). aCg = anterior cingulate cortex; DR/MnR = dorsal and median raphe nuclei; GPh = human equivalent of habenula-projecting globus pallidus; IPN = interpeduncular nucleus; LC = locus coeruleus; DLTg = laterodorsal tegmental nucleus; LH = lateral hypothalamus; LHb = lateral habenular complex; LPO = lateral preoptical area; MHb = medial habenular complex; NI = nucleus incertus; sCg = subgenual cingulate cortex; septum = triangular septal nucleus and the bed nuclei of the anterior commissure; PHC = parahippocampal cortex; RMTg = rostromedial tegmental nucleus; VTA = ventral tegmental area.
A phenomenon that was not discussed in this way previously is the co-release of glutamate and GABA by somatostatin-containing neurons projecting from the entopeduncular nucleus to the lateral habenula (Shabel et al., Reference Shabel, Proulx, Piriz and Malinow2014; Wallace et al., Reference Wallace, Saunders, Huang, Philson, Goldman, Macosko, McCarroll and Sabatini2017; Root et al., Reference Root, Zhang, Barker, Miranda-Barrientos, Liu, Wang and Morales2018), as well as by projections from the VTA to the lateral habenula (Root et al., Reference Root, Zhang, Barker, Miranda-Barrientos, Liu, Wang and Morales2018) and the co-release of acetylcholine and glutamate by medial habenular neurons projecting to the interpeduncular nucleus (Ren et al., Reference Ren, Qin, Hu, Tan, Qiu, Zhao, Feng and Luo2011). This co-release of GABA and glutamate would open the possibility of modulating the balance between excitation and inhibition and thus may be important in cocaine dependence (Meye et al., Reference Meye, Soiza-Reilly, Smit, Diana, Schwarz and Mameli2016) and the emergence of depression (Shabel et al., Reference Shabel, Proulx, Piriz and Malinow2014). It has been suggested that habenular glial cells (astrocytes) determine the balance between activities by changing the micro-environment (Aizawa & Zhu, Reference Aizawa and Zhu2019; Metzger et al., Reference Metzger, Souza, Lima, Bueno, Gonçalves, Sego, Donato and Shammah-Lagnado2021). It is possible that the interaction between astrocytes and microglia also plays a role here (Agostinho et al., Reference Agostinho, Madeira, Dias, Simões, Cunha and Canas2020), but this interaction within the habenula has, to date, barely been studied.
More attention could be paid to the bidirectional nature of the connections between the lateral habenula and the upper brainstem, although this greatly increases the complexity (Metzger et al., Reference Metzger, Souza, Lima, Bueno, Gonçalves, Sego, Donato and Shammah-Lagnado2021).
Circuits regulating pleasure and happiness
When the theoretical backgrounds of the depressive mood disorders are summarised, the picture emerges that actually two characteristic components can be distinguished (Loonen & Ivanova, Reference Loonen and Ivanova2016a, Reference Loonen and Ivanova2016b). Depression is characterised on the one hand by lack of energy, lack of motivation and anhedonia and on the other by worrying, feelings of helplessness and of hopelessness. These two components are linked and cannot be completely distinguished, but they can vary in severity independently of each other. The extrapyramidal system generally has the function of controlling the intensity of the output; the dorsal division that of motor skills and cognitive performance, and the ventral division that of addiction and the flight response. The ventral striatum (also called nucleus accumbens) can be seen as the interface of the limbic and motor/cognitive part of the forebrain (Groenewegen, Reference Groenewegen2007). The core portion (NAcbC) is part of a set of cortico-striato-thalamo-cortical (CSTC) circuits, which, for example in addiction, regulate the intensity of (reinstatement of) drug-seeking behaviour (Kalivas & McFarland, Reference Kalivas and McFarland2003) and the shell part (NacbS) of, among other things, hyperkatifeia in the negative affect stage (Koob, Reference Koob2021).
Based on these data, we developed a model for the neurobiological backgrounds of the processes that cause the two components of depressive disorder. In the model presented, the role of the NAcbC and NAcbS were oversimplified to illustrate regulating reward-seeking and distress-avoiding behaviour, respectively. We also postulate the existence of two re-entry CSTC circuits: one with the start and end in the ventral part of the pregenual anterior cingulate cortex (aCg) and the other in the subgenual anterior cingulate cortex (sCg) (Loonen & Ivanova, Reference Loonen and Ivanova2016a). We hypothesised that when the reward-seeking behaviour stimulated by the first group of CSTC circuits is successful, the individual experiences pleasure, while the success of the second type of distress-avoiding behaviour results in feelings of happiness. We have named them as circuits regulating pleasure and happiness accordingly. The activity of the CSTC circuits is regulated by ascending dopaminergic, serotonergic and adrenergic pathways from the upper brainstem (Loonen & Ivanova, Reference Loonen and Ivanova2016a, Reference Loonen and Ivanova2016b, Reference Loonen and Ivanova2016c). The activity of the latter are, in turn, controlled by the habenula.
Putative involvement of habenular physiology in (neuro)psychiatric disorders
It should have become clear that we have good reasons to believe that dorsal pathways relaying within the habenula may play an important role in addiction, anxiety, compulsion, depression, mania and psychosis (Loonen & Ivanova, Reference Loonen and Ivanova2018a for an overview). For example, there is now ample evidence from imaging studies in humans, as well as from a large number of animal studies, that a hyperactive lateral habenula may play a prominent role in the pathophysiology of major depressive disorder and other stress-related psychiatric disorders (see Metzger et al., Reference Metzger, Souza, Lima, Bueno, Gonçalves, Sego, Donato and Shammah-Lagnado2021). The importance of the lateral habenula in the therapy of mental disorders is perhaps most apparent by the results of deep brain stimulation of the habenula because this treatment appears to be casuistically effective in treatment-refractory depression, obsessive-compulsive disorder and schizophrenia (Sartorius et al., Reference Sartorius, Kiening, Kirsch, von Gall, Haberkorn, Unterberg, Henn and Meyer-Lindenberg2010; Kiening & Sartorius, Reference Kiening and Sartorius2013; Wang et al., Reference Wang, Zhang, Zhang, Gong, Li, Jin, Li, Liu and Sun2020, Reference Wang, Cai, Qiu, Yao, Tian, Gong, Zhang, Xu, Zhang, Zang, Liu, Peng and Li2021; Zhang et al., Reference Zhang, Zhang, Li, Deng, Nuttin, Voon and Sun2020). In addition, treatment of depression with intravenous administration of ketamine – an NDMA antagonist known to affect the functioning of the habenula – affects the functional connectivity of this complex (Rivas-Grajales et al., Reference Rivas-Grajales, Salas, Robinson, Qi, Murrough and Mathew2021). However, the distinct connections of the (more than 15) different habenula subnuclei are organised in far too complex a way to allow fruitful analysis of the underlying mechanisms to explain these findings. In addition, major depressive disorder (Loonen & Ivanova, Reference Loonen and Ivanova2016b; Duman et al., Reference Duman, Sanacora and Krystal2019), obsessive-compulsive disorder (Vlček et al., Reference Vlček, Polák, Brunovský and Horáček2018; Loonen & Ivanova, Reference Loonen and Ivanova2019b) and schizophrenia (Owen et al., Reference Owen, Sawa and Mortensen2016; Guan et al., Reference Guan, Ni, Zhu, Williams, Cui, Li, Tubbs, Sham and Gui2021) are composite disorders which are likely to be simultaneously caused by multiple neurobiological disturbances to a mutually variable extent. The role of the habenula in the symptoms of neuropsychiatric disorders based on non-congenital brain damage has been little investigated. In rodents, the (lateral) habenula has been found to play a role in the stress sensitivity of reward-related cognitive assessments (Nuno-Perez et al., Reference Nuno-Perez, Trusel, Lalive, Congiu, Gastaldo, Tchenio, Lecca, Soiza-Reilly, Bagni and Mameli2021), in deliberate self-harm (Guo et al., Reference Guo, Tang, Zhang, Jin, Li, Ding, Zhang, Yang, Zhou, He, Xu, Bi, Xu and Lau2018) and in aggression (Golden et al., Reference Golden, Heshmati, Flanigan, Christoffel, Guise, Pfau, Aleyasin, Menard, Zhang, Hodes, Bregman, Khibnik, Tai, Rebusi, Krawitz, Chaudhury, Walsh, Han, Shapiro and Russo2016; Flanigan et al., Reference Flanigan, Aleyasin, Takahashi, Golden and Russo2017), all of which are phenomena commonly seen in neuropsychiatric disorders. The contribution of the habenula to avolition, apathy and anhedonia can be expected, based on its contribution to depressive behavior (Loonen & Ivanova, Reference Loonen and Ivanova2016a; Aizawa & Zhu, Reference Aizawa and Zhu2019). It is, therefore, pertinent to further investigate the role of the habenula in the pathogenesis of these and other symptoms of neuropsychiatric syndromes.
Application of these theories to develop new biomarkers
Unravel the different mechanisms behind causes of mental illness
In the search for suitable biomarkers, an important problem is encountered by the way in which we consider and classify mental disorders. Mental illnesses are not comparable to infectious diseases, which have a specific pathogen that can be identified and combated with antibiotics. They should not even be compared with a disease like pneumonia, which can be caused by several pathogens, but which all produce a more or less uniform clinical picture. We want to defend the idea that most mental disorders involve one or more biological disturbances that are not sufficient in themselves to cause the disorder, but which, in combination with predisposition, development and environmental factors, prevent the individual from breaking free from a vicious circle of abnormal behaviour and stream of thoughts. For example, the same feeling of insecurity can lead to delusions of persecution in some people and to depressed feelings of helplessness and hopelessness in others. On the other hand, a similar paranoid delusion may have been caused in one person by a basic feeling of insecurity and in another by a constant distortion of perceptions. To this, it can be added that humans also have the ability to think and reason and that this thinking does not depend on the structure of the biological hardware. This biologically unbound mind determines the natural capacity for recovery with which an individual can keep his behavior on track despite biological disturbances. These insights lead to the belief that unified biomarkers for schizophrenia or depression probably do not exist. It is better for us to look for biomarkers for specific biological functions such as motivation for reward-seeking behaviour or attentive salience, which can also be an entry point to treat various mental disorders.
The functioning of the habenuloid conduction system can be used well as a starting point in identifying suitable biomarkers: until now, little attention has been paid to this. We must currently, therefore, suffice with the interpretation of the results of the research into the role of biochemical entities in the context of this conduction system which thereby suggests possible application as biomarkers.
Considering the connectivity via the habenuloid complex
The vast majority of the habenuloid complex output to midbrain nuclei is glutamatergic in nature, either primarily or as a co-transmitter. This is not actually directly useful for identifying potential biomarkers because the glutamatergic system is widespread in the brain and the targets of the habenula are also affected by glutamatergic fibres from other parts of the forebrain. It is therefore better to look at the starting point of these projections in the habenuloid complex itself.
The rapid antidepressant action of ketamine is the main argument for the involvement of the glutamatergic system of the habenuloid complex. Ketamine is a non-competitive N-methyl-D-aspartate (NMDA) receptor antagonist and affects the functioning of the (lateral) habenula (Carlson et al., Reference Carlson, Diazgranados, Nugent, Ibrahim, Luckenbaugh, Brutsche, Herscovitch, Manji, Zarate and Drevets2013; Shepard et al., Reference Shepard, Langlois, Browne, Berenji, Lucki and Nugent2018; Yang et al., Reference Yang, Cui, Sang, Dong, Ni, Ma and Hu2018). On closer examination, however, the relationship turns out to be considerably more ambiguous than expected. Firstly, there are doubts about whether NMDA antagonism is the explanation for the antidepressant effect of ketamine (Hashimoto, Reference Hashimoto2020). Ketamine is a racemic mixture and the R-form and its metabolite (2R,6R)-hydroxynorketamine have much less affinity to NMDA receptors, but have a good antidepressant effect, at least in animal models, as in a pilot study of R-ketamine (Hashimoto, Reference Hashimoto2020; Jelen et al., Reference Jelen, Young and Stone2021; Leal et al., Reference Leal, Bandeira, Correia-Melo, Telles, Mello, Vieira, Lima, Jesus-Nunes, Guerreiro-Costa, Marback, Caliman-Fontes, Marques, Bezerra, Dias-Neto, Silva, Sampaio, Sanacora, Turecki, Loo, Lacerda and Quarantini2021). Secondly, ketamine is originally a dissociative anaesthetic and its pharmacological effects at sub-anaesthetic doses may also be related to its narcotic or psychotomimetic effects (Inserra et al., Reference Inserra, De Gregorio and Gobbi2021). Thirdly, ketamine has many other effects that may contribute to its antidepressant activity (Zanos & Gould, Reference Zanos and Gould2018; Athira et al., Reference Athira, Mohan and Chakravarty2020; Lisek et al., Reference Lisek, Zylinska and Boczek2020; Asim et al., Reference Asim, Wang, Hao and Wang2021). Fourthly, NMDA receptors are found in many different places in the brain and on several cellular elements in the habenula (Gold & Kadriu, Reference Gold and Kadriu2019; Lusher et al., Reference Luscher, Feng and Jefferson2020; Stenovec et al., Reference Stenovec, Li, Verkhratsky and Zorec2020; Jelen et al., Reference Jelen, Young and Stone2021). In recent years, more attention has been paid to the slow neuroplastic changes initiated by ketamine administration (Lisek et al., Reference Lisek, Zylinska and Boczek2020). This mainly focuses on the activation of the mammalian Target Of Rapamycin (mTOR) pathway in the prefrontal cortex (Jelen et al., Reference Jelen, Young and Stone2021), which appears to be related to the release of brain-derived neurotropic factor (Lisek et al. Reference Lisek, Zylinska and Boczek2020). It is not known to us whether this interaction between the glutamatergic and GABAergic system resulting in mTOR activation also occurs within the habenula. That is also not necessary, because there are several other ways in the habenula by which the activity of efferent glutamatergic neurons can be adjusted. In this context, it makes sense to pay attention to the regulation via habenular astroglial cells (Stenovec et al., Reference Stenovec, Li, Verkhratsky and Zorec2020; Frizzo & Ohno, Reference Frizzo and Ohno2021) and other neuroglia (Sancho et al., Reference Sancho, Contreras and Allen2021). In the physiology of astrocytes, several components of the regulation of glutamatergic neurotransmission converge, which may play a role in the pathogenesis of (neuro)psychiatric disorders (Blanco-Suárez et al., Reference Blanco-Suárez, Caldwell and Allen2017). Via transporter proteins, astrocytes regulate perisynaptic glutamate and potassium concentrations (Frizzo & Ohno, Reference Frizzo and Ohno2021). It should be noted that some variants of the NMDA receptor can be activated by glycine alone (Otsu et al., Reference Otsu, Darcq, Pietrajtis, Mátyás, Schwartz, Bessaih, Abi Gerges, Rousseau, Grand, Dieudonné, Paoletti, Acsády, Agulhon, Kieffer and Diana2019; Stroebel et al., Reference Stroebel, Mony and Paoletti2021). The amino acid glycine is secreted by astrocytes, which can also regulate extracellular concentration through reuptake (Harsing & Matyus, Reference Harsing and Matyus2013). Not much is known about the preference for the dorsal diencephalic pathway. In interaction with microglia, astrocytes also play an important role in mediating neuroinflammation (D’Antoni et al., Reference D’Antoni, Berretta, Bonaccorso, Bruno, Aronica, Nicoletti and Catania2008; Pinto et al., Reference Pinto, Passos, Librenza-Garcia, Marcon, Schneider, Conte, da Silva, Lima, Quincozes-Santos, Kauer-Sant and Kapczinski2018). They express metabotropic glutamate (mGlu) receptors and are thus involved in neurodegenerative processes (Spampinato et al., Reference Spampinato, Copani, Nicoletti, Sortino and Caraci2018). It can also be mentioned that the mGlu2 receptor with which the medial habenula shows an increased binding (Wright et al., Reference Wright, Johnson, Zhang, Salhoff, Kingston, Calligaro, Monn, Schoepp and Marek2013) is barely present on astrocytes but is present on microglia (Spampinato et al., Reference Spampinato, Copani, Nicoletti, Sortino and Caraci2018). Microglia can be considered to be a rising star in the pathogenesis of depression (Deng et al., Reference Deng, Chen and Wang2020; Jia et al., Reference Jia, Gao and Hu2021) and many other inflammatory and non-inflammatory conditions of the central nervous system (Brisch et al., Reference Brisch, Wojtylak, Saniotis, Steiner, Gos, Kumaratilake, Henneberg and Wolf2021; Rahimian et al., Reference Rahimian, Wakid, O’Leary and Mechawar2021). What part of this is mediated by influencing the DDC system has, to our knowledge, been the subject of very little study as yet.
A considerably less well-investigated area of possible habenular neuro-glial regulation is found in the area of purinergic neurotransmission (for review see Agostinho et al, Reference Agostinho, Madeira, Dias, Simões, Cunha and Canas2020). It has been found that the purines ATP and ADP are released from an isolated rat habenula preparate upon axonal stimulation (Sperlágh et al, Reference Sperlágh, Kittel, Lajtha and Vizi1995) and that astrocytes of the medial habenula express on their cell surface the enzyme ectonucleoside riphosphate diphosphohydrolase 2 (NTPDase2), which hydrolyses extracellular ATP (Gampe et al., Reference Gampe, Hammer, Kittel and Zimmermann2012). This supplementary astrocytic regulatory system modulates direct purinergic input to neuronal synapses. Purinergic neurotransmission may play an important role in neuroplastic and neuronal degeneration such as occurs in depression (Ribeiro et al., Reference Ribeiro, Roncalho, Glaser, Ulrich, Wegener and Joca2019).
A significant part of the dorsal diencephalic connectivity between the forebrain and midbrain via the medial habenula is by cholinergic neurotransmission, in particular by activation of nicotinic acetylcholine (AChN) receptors (see Antolin-Fontes et al., Reference Antolin-Fontes, Ables, Görlich and Ibañez-Tallon2015 for review). Although this somewhat belies the complexity of the organisation of the medial habenula into a large number of sub-nuclei and sub-regions and the associated intricacy of input and output connectivity, we want to simplify the description drastically according to Antolin-Fontes et al. (Reference Antolin-Fontes, Ables, Görlich and Ibañez-Tallon2015). From this point of view, it is worth noting that post-synaptic AChN receptors play a role in processing input to the medial habenula from the posterior septum and from the medial habenula to interpeduncular nucleus, while presynaptic AChN receptors are present on medial habenular glutamatergic fibres running to the interpeduncular nucleus (Antolin-Fontes et al., Reference Antolin-Fontes, Ables, Görlich and Ibañez-Tallon2015). In the latter fibres, acetylcholine is a co-transmitter. It should be noted that although the medial habenula is also the main source of the input of the IPN, cholinergic fibres may also originate in the posterior septum directly (Contestabile & Fonnum, Reference Contestabile and Fonnum1983; Fonnum & Contestabile, Reference Fonnum and Contestabile1984) and (although disputed by Contestabile & Fonnum Reference Contestabile and Fonnum1983) in the diagonal band of Broca (Gottesfeld & Jacobowitz, Reference Gottesfeld and Jacobowitz1978; Albanese et al., Reference Albanese, Castagna and Altavista1985). Within the context of this review, it is interesting to look at the composition of AChN receptors present (Antolin-Fontes et al., Reference Antolin-Fontes, Ables, Görlich and Ibañez-Tallon2015). The neuronal system formed by the medial habenula and interpeduncular nucleus is highly enriched with α5, α3 and β4 subunits. Other subunits are present but less specific. It may therefore be interesting to genotype people for genes encoding of these subunits in order to gain insight into the sensitivity to activation of this pathway in neuropsychiatric disorders other than those associated with nicotine dependence and withdrawal.
Two other neurotransmitters that play a dominant role in dorsal diencephalic connectivity are GABA and substance P. This may involve co-transmission with, for example, glutamate (Antolin-Fontes et al., Reference Antolin-Fontes, Ables, Görlich and Ibañez-Tallon2015; Zahm & Root, Reference Zahm and Root2017). At least as far as substance P is concerned, this mainly involves the medial habenula and a relatively small medial area of the lateral habenula (Antolin-Fontes et al., Reference Antolin-Fontes, Ables, Görlich and Ibañez-Tallon2015; Zahm & Root, Reference Zahm and Root2017). Of interest is the high expression of the GABAB receptor in the medial habenula (Durkin et al., Reference Durkin, Gunwaldsen, Borowsky, Jones and Branchek1999; Princivalle et al., Reference Princivalle, Regondi, Frassoni, Bowery and Spreafico2000; Charles et al., Reference Charles, Evans, Robbins, Calver, Leslie and Pangalos2001) and the availability of baclofen as a centrally active GABAB agonist which can be used as a probe. The presynaptic GABAB receptor plays a role in reducing the activity of GABAergic synapses by long-term depression (GABA LTD) (Lalive et al., Reference Lalive, Nuno-Perez, Tchenio and Mameli2022). Habenular GABAB receptors could play a role in processing chronic mild stress exposure along these lines on regulating the balance between GABAergic and glutamatergic inputs; this could play a role in stress-related psychiatric disorders. For the neurokinin 1 receptor, which mediates the effects of substance P, any research and data have not yet been sufficiently elaborated. The same can be said about the role of receptors for μ- and κ-opioid peptides and cannabinoids (CB1), which, similar to substance P (Yang et al., Reference Yang, Yu, Jin and Zhao2014; Lee et al., Reference Lee, Lee, Woo, Kang, Kim Kwon and Shin2018), also produce interesting effects via the dorsal diencephalic trajectory (Gardon et al., Reference Gardon, Faget, Chu Sin Chung, Matifas, Massotte and Kieffer2014; Boulos et al., Reference Boulos, Ben Hamida, Bailly, Maitra, Ehrlich, Gavériaux-Ruff, Darcq and Kieffer2020; Simmons et al., Reference Simmons, Shepard, Gouty, Langlois, Flerlage, Cox and Nugent2020; Vickstrom et al., Reference Vickstrom, Liu, Liu, Hu, Mu, Hu, Yu, Love, Hillard and Liu2021; Zapata & Lupica, Reference Zapata and Lupica2021). We have recently obtained evidence that serum levels of β-endorphin, an endogenous μ- and δ-opioid receptor agonist (Terenius, Reference Terenius2000), are lower in patients with alcohol use disorder, particularly in those with depressive co-morbidity and correlate with physical aggression (Roschina et al., Reference Roschina, Levchuk, Boiko, Michalitskaya, Epimakhova, Losenkov, Simutkin, Loonen, Bokhan and Ivanova2021). As some fibres from the arcuate nucleus, expressing the precursor protein of β-endorphin, pass through the stria medullaris to the medial habenula (Sim & Joseph, Reference Sim and Joseph1991) and the serum levels may express the activity of intracerebral release, it is tempting to speculate that this might have something to do with changes in μ-opioid receptors activation in the medial habenula.
We attach great importance to the possible role of signalling by habenula-projecting globus pallidus (GPh) neurons. The significance of these glutamatergic neurons has been investigated and demonstrated especially in the most primitive vertebrates, the lamprey. In our opinion, further mapping of the possible equivalents of these neurons in humans and further characterisation with regard to co-transmitters, receptor subtypes, intracellular proteins and effectors should be given high priority (Loonen & Ivanova, Reference Loonen and Ivanova2018b).
The lateral habenula not only gives output to the monoaminergic nuclei in the midbrain, but also receives input from the dopaminergic substantia nigra and VTA, the adrenergic locus coeruleus and the serotonergic raphe nuclei (Batalla et al., Reference Batalla, Homberg, Lipina, Sescousse, Luijten, Ivanova, Schellekens and Loonen2017). We became particularly interested in dopamine D4 receptors (DRD4) after finding that variants of the DRD4 gene were associated with the magnitude of response to antidepressants (Ochi et al., Reference Ochi, Vyalova, Losenkov, Paderina, Pozhidaev, Loonen, Simutkin, Bokhan, Wilffert and Ivanova2021). Dopamine D4 receptors in the lateral habenula are associated with depressive responses (Hui et al., Reference Hui, Du, Xu, Zhang, Tan and Liu2020) and these receptors may also be associated with relapse to nicotine, cocaine and amphetamine use in addiction (Di Ciano et al., Reference Di Ciano, Grandy and Le Foll2014). Because dopamine D4 receptors in the habenula are stimulated by norepinephrine released from adrenergic terminals and not dopamine (Root et al., Reference Root, Hoffman, Good, Zhang, Gigante, Lupica and Morales2015), this could also link to the antidepressant and other psychotropic effects of indirectly acting adrenergic agonists such as tricyclic antidepressants.
Neuroinflammation may also play an important role in the development of depression (Loonen & Ivanova, Reference Loonen and Ivanova2016b). It has been suggested that this is related to the remodelling of the extracellular matrix – and therefore modulating the function – of the lateral habenula (Ito et al., Reference Ito, Nozaki, Sakimura, Abe, Yamawaki and Aizawa2021). The release of cytokines from microglia and other immunocompetent cells in the lateral habenula probably plays an important role in the generation of a depressive response (Zhao et al., Reference Zhao, Pan, Tang and Lin2018; Guan et al., Reference Guan, Huang, Xu, Gao, Lin, Huang, Wang, Li, Wu, Yao, Wang, Zhang, Teoh, Xuan and Sun2020; Ito et al., Reference Ito, Nozaki, Sakimura, Abe, Yamawaki and Aizawa2021). It is likely that the same process plays a role in morphine withdrawal (Valentinova et al., Reference Valentinova, Tchenio, Trusel, Clerke, Lalive, Tzanoulinou, Matera, Moutkine, Maroteaux, Paolicelli, Volterra, Bellone and Mameli2019). Interestingly, interleukin 6 and interleukin 18 are also expressed neuronally in the habenula (Schöbitz et al., Reference Schöbitz, Voorhuis and De Kloet1992; Sugama et al., Reference Sugama, Cho, Baker, Joh, Lucero and Conti2002). There is, therefore, every reason to consider the relationship between cytokines, depression and habenula functioning from two perspectives: as a biomarker for habenula functioning and as a confounder when examining other measures.
The superfamily of G-protein-coupled receptors includes a large group of trans-membrane receptors, which achieve their action by activating so-called G-proteins (Kristiansen, Reference Kristiansen2004). GPCRs include receptors for a large number of ligands such as, for example, neurotransmitters, neuromodulators and hormones. However, a substantial number of receptors have also been identified for which the endogenous ligand is still unknown: so-called Orphan GCPRs. Some of these are concentrated in the habenula and might therefore play a role in the development of reward- and stress-related psychiatric disorders (Roy & Parhar, Reference Roy and Parhar2022). As an example, we mention GPR151 whose gene is abundantly expressed in the medial and lateral habenula (Broms et al., Reference Broms, Antolin-Fontes, Tingström and Ibañez-Tallon2015). After establishing their relevance for these disorders in cross-sectional or longitudinal genotyping experiments, newly developed ‘designer’ drugs with affinity for such Orphan GCPRs could serve to map their biological significance in animal studies and to investigate their utility as biomarkers.
There is, of course, a whole series of proteins that are (also) localised in the dorsal diencephalic pathway and play a role in its activity as receptor, effector or indirect modulator. In theory, these could all potentially be developed as biomarkers of function. We have previously advocated paying close attention to the phylogenetic development within the system (Pérez-Fernández et al., Reference Pérez-Fernández, Megías and Pombal2016) when developing new treatments and suitable biomarkers (Loonen & Ivanova, Reference Loonen and Ivanova2018b). The same can be said about its ontogenetic development (Schmidt & Pasterkamp, Reference Schmidt and Pasterkamp2017). However, there is still a long way to go in these areas.
Conclusions
The most promising biomarkers are those linked to activation or inhibition of the nicotine receptor, DRD4 receptor, μ-opioid receptor and the functioning of habenular glia cells (astrocytes and microglia). The glutamatergic and GABAergic neurotransmitter systems also offer many opportunities for the development of biomarkers, but the lack of specificity for the dorsal diencephalic pathway is still a barrier. The search for and characterisation of components preferentially present in the habenula and involved in the development of (neuro)psychiatric disorders deserve, in our opinion, a high priority. However, the animal models for mental disorders used in much of the research described have major limitations and significant species differences may exist. Moreover, the habenula is complexly organised and plays a role in many physiological processes, so that the effects observed in the laboratory animal may often have come about indirectly.
Future perspectives
The most encouraging method to investigate (combinations of) biomarkers that tell something about the functioning of the medial or lateral habenula as a component within the dorsal diencephalic trajectory is to measure the response of the concentration of these biomarkers after administration of a receptor agonist or antagonist in individuals who carry more or less active genetic variants of this receptor. This type of research should be preceded by studying key mechanisms of habenular functioning in order to identify the receptors to be further investigated. Experimental research in laboratory animals is hypothesis-generating, despite all its limitations.
The search for variants of the NMDA receptor with a certain specificity for the dorsal diencephalic pathway is, in our opinion, a high priority. The previously mentioned specificity for being stimulated by glycine is one of them (Otsu et al., Reference Otsu, Darcq, Pietrajtis, Mátyás, Schwartz, Bessaih, Abi Gerges, Rousseau, Grand, Dieudonné, Paoletti, Acsády, Agulhon, Kieffer and Diana2019; Stroebel et al., Reference Stroebel, Mony and Paoletti2021). The NMDA receptor is usually a heterotetramer of NR1, NR2 and/or NR3 subunits (Bai & Hoffman, Reference Bai, Hoffman and van Dongen2009). The Grin1, Grin2 and Grin3 genes code for these subunits. It might be valuable to investigate whether some parts of the dorsal diencephalic connectivity are sensed by specific NMDA subtypes, just as it is the case for the nicotinic cholinergic receptor (Antolin-Fontes et al., Reference Antolin-Fontes, Ables, Görlich and Ibañez-Tallon2015).
As previously indicated, it is best to look closely at the phylogenetic development of the mechanisms involved: an example might be the role of the dopamine D4 receptor. The dopamine D4 receptor in mammals has a distribution that differs significantly from that of dopamine D2 and D3 receptors (Primus et al., Reference Primus, Thurkauf, Xu, Yevich, McInerney, Shaw, Tallman and Gallagher1997; Beaulieu & Gainetdinov, Reference Beaulieu and Gainetdinov2011). Apart from being generally lower in density, the dopamine D4 receptor is not found in the ventral (accumbens) and dorsal (caudate, putamen) striatum (Primus et al., Reference Primus, Thurkauf, Xu, Yevich, McInerney, Shaw, Tallman and Gallagher1997). This is because these structures arose much later during the evolution of vertebrates (Loonen & Ivanova, Reference Loonen and Ivanova2015, Reference Loonen and Ivanova2016c). In the lamprey striatum (animals which correspond to the earliest ancestors among vertebrates), dopamine D4 receptors are found in cells representing a subpopulation within this nucleus (Pérez-Fernández et al., Reference Pérez-Fernández, Megías and Pombal2016). The forebrain of these earliest vertebrate ancestors later in evolution became the amygdaloid complex (Loonen & Ivanova, Reference Loonen and Ivanova2015, Reference Loonen and Ivanova2016c). Within the context of this review, it is important to note that agonists and antagonists of the dopamine D4 receptors have effects within the amygdaloid complex and the lateral habenula (Hui et al., Reference Hui, Du, Xu, Zhang, Tan and Liu2020), but less so within the ventral and dorsal striatum.
We would like to emphasise that we do not believe in the existence of biomarkers for categories of mental disorders. It is better to look for biomarkers that are linked to concrete psychological dysfunctions and as such contribute to (the genesis of) (neuro)psychiatric syndromes. Embedding research in the Research Domain Criteria (RDoC) framework of the NIMH can be enlightening in this regard (National Institute of Mental Health, 2009).
Data availability statement
Not applicable.
Acknowledgements
The authors greatly appreciate the help of Kate Barker (BA, PGCE) who proofread the manuscript. This work was carried out within the framework of Tomsk Polytechnic University Competitiveness Enhancement Program. Dutch universities have suspended all forms of cooperation with Russian educational and research institutions due to the conflict with Russia over Ukraine. The Board of the Faculty of Science and Engineering of the University of Groningen exempted the manuscript of this article because it had already been submitted, reviewed and revised before this decision.
Author contributions
A.L. developed the ideas and wrote the manuscript. S.I. discussed the ideas and commented on the manuscript. A.L. thereafter finalised the manuscript.
Financial support
This research did not receive any specific grant from funding agencies in the public, commercial or not-for-profit sectors.
Conflict of interest
None.