Introduction
Cellular plasticity describes the ability of certain cells to adopt different phenotypes and functions, which is a characteristic feature of embryonic stem cells. However, such trait has also been observed in adult differentiated cells when challenged by chronic physiological or pathological conditions such as wound repair and tumourigenesis (Ref. Reference Yuan, Norgard and Stanger1). In cancer, cell plasticity endows tumours with enhanced self-renewal and pro-invasiveness capacities. Further, by attaining different phenotypes, tumour cells can bypass cell cycle arrest and apoptosis and circumvent therapeutic insults (Ref. Reference Boumahdi and de Sauvage2). Indeed, tumour microenvironment (TME) plays a crucial role in fuelling tumour plasticity by exposing tumour cells to a wide variety of stimuli from a heterogeneous niche, thereby imposing a significant obstacle in cancer management (Refs Reference Yuan, Norgard and Stanger1–Reference da Silva-Diz3).
Head and neck squamous cell carcinoma (HNSCC) represents a group of common and aggressive epithelial tumours that arise in the oral cavity (oral SCC; OSCC), oropharynx and larynx. These tumours have strong associations with smokeless and smoking tobacco products, betel chewing, alcohol dependence and infection with human papillomavirus (HPV) types 16 and 18 (Ref. Reference Johnson4). Recently, a shift in the composition and relative abundance of oral microbiota (i.e. oral dysbiosis) has also been linked to HNSCC and certain lesions associated with an increased risk of OSCC known as potentially malignant disorders (Ref. Reference Metsäniitty5). Owing to their invasiveness and high metastatic potential, HNSCC accounted for 878 348 new cases and 444 347 new deaths in 2020 alone (Ref. Reference Sung6). Currently, treatment options include surgery, chemo-radiation, targeted therapy, immunotherapy or a combination of these modalities. Despite marked improvement in cancer management, metastasis and drug resistance remain the main causes of deaths in these patients. Although survival can be prolonged with a multimodal approach, this may, however, induce drug toxicity and deteriorate the patients' quality of life. Thus, the 5-year survival rate remains stagnant at approximately 50% (Refs Reference Johnson4, Reference Xing7, Reference Muzaffar8).
Recent technical advances in single-cell genomics, such as single-cell RNA sequencing (scRNA-seq), have revealed the complexity and heterogeneity of HNSCC, which influence both tumour plasticity and clinical response. Within such a diverse ecosystem, tumour cells exhibit variable expression of signatures related to cell cycle, stress, epithelial-to-mesenchymal transition (EMT), hypoxia and epithelial differentiation (Ref. Reference Puram9). Importantly, a distinct subpopulation of cancer stem (or stem-like) cells (CSCs) exists with enhanced self-renewal and protumourigenic properties in multiple cancers, including HNSCC (Fig. 1) (Refs Reference Prince10–Reference Biddle12). It is thus critical to understand the behaviour of these dynamic cells to develop more effective anticancer therapies. Herein, we appraise the current knowledge of CSCs in HNSCC, highlighting some recently identified mechanisms that mediate their phenotypic plasticity and immune evasion. We also discuss critical factors governing their interplay within TME, including our evolving appreciation of the contribution of oral microbiota.

Fig. 1. Cancer stem cells (CSCs) in head and neck squamous cell carcinoma. CSCs represent a small subpopulation of tumour cells with the following main features: (a) self-renewal, (b) transdifferentiation and phenotypic switching, (c) tumour initiation (tumourigenesis) when transplanted into an animal host, (d) high invasive and metastatic potential, and (e) ability to develop anti-tumour drug resistance.
Intratumoural heterogeneity in HNSCC
Given the limited success of traditional therapies, attention has also been focused on identifying the genotype variations between cancer patients to predict their response to targeted drugs. However, TME harbours, within the same patient, subpopulations of tumour cells with different phenotypes and mutations, referred to as intratumoural heterogeneity (Ref. Reference Bedard13). Intratumoural heterogeneity drives clinical resistance and poses a major challenge for designing effective therapies in HNSCC (Refs Reference Bedard13, Reference Canning14). Initially, two models were proposed to explain intratumoural heterogeneity. On the one hand, the stochastic model of clonal evolution postulates that every tumour cell with an appropriate set of somatic mutations can initiate and sustain a ‘metastable’ tumour growth. However, this model conceives tumours as a homogeneous mass, hence falling short of explaining the variations in tumourigenic potential and multidrug resistance. On the other hand, according to the hierarchical cell model, cancer initiation and progression are driven mainly by a subpopulation of dynamic cells – CSCs – that are intrinsically different from the majority of more differentiated tumour cells (Refs Reference Shackleton15, Reference Fanelli, Naccarato and Scatena16).
In essence, CSCs were termed as such to highlight their stem cell-like properties, including their self-renewal, transdifferentiation and migration abilities. In this regard, there has been overwhelming evidence supporting this ‘stemness’ model. CSCs were first characterised as a minority of CD34+ve/CD38−ve cells in acute myeloid leukaemia (Ref. Reference Lapidot17). Thereafter, CSCs with other surface markers have been identified in different cancers. For instance, CD44+ve and CD133+ve CSCs sustained the capacity to initiate new tumours in non-obese diabetic/severe combined immunodeficient (NOD/SCID) mouse models of breast and colorectal cancers, respectively (Refs Reference Al-Hajj18, Reference O'Brien19). The first identification of CSCs in HNSCC was reported by Prince et al., who showed that only CD44+ve cancer cells – comprising <10% of the tumours – initiated new malignant growths in NOD/SCID mice (Ref. Reference Prince10). Interestingly, as few as 5 × 103 cells of early-passaged CD44+ve CSCs were able to produce new tumours in vivo, whereas CD44−ve cells failed to give rise to tumour events at a 100-fold higher density. Of note, CD44+ve cell-derived tumours comprised phenotypically diverse cells of both CD44+ve/CD44−ve clones, suggesting that CSCs may also drive intratumoural heterogeneity in HNSCC patients. These findings signify the role of CD44 in identifying CSCs in other tumours of epithelial origin (Ref. Reference Prince10). Several cell surface receptors and intracellular proteins have since been reported as applicable CSC markers in HNSCC, as summarised in Table 1.
Table 1. Common markers of cancer stem cells in head and neck cancers
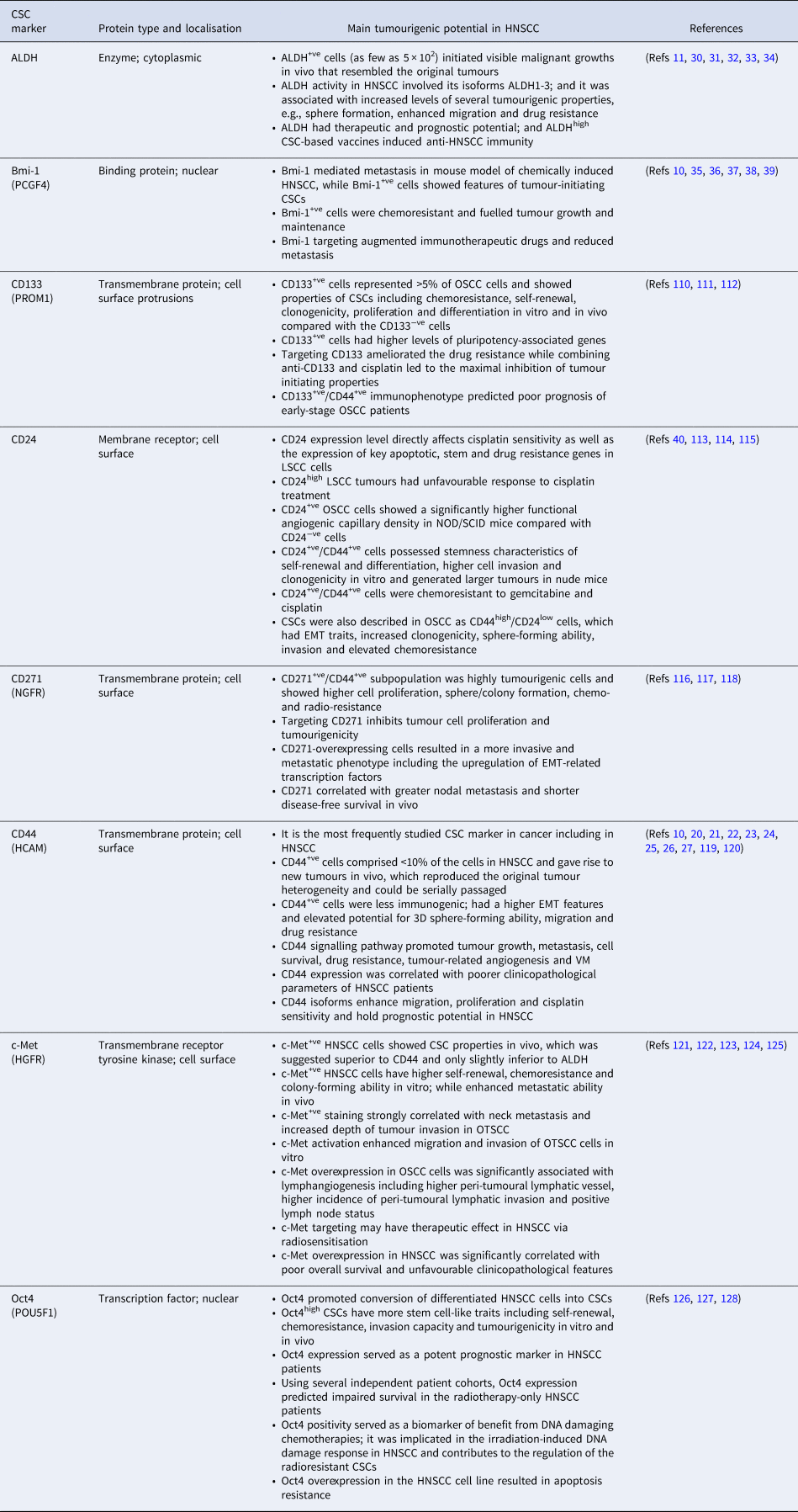
3D, three-dimensional; ALDH, aldehyde dehydrogenase; Bmi-1, B-cell-specific Moloney murine leukaemia virus insertion site 1; CD, cluster of differentiation; c-Met, c-mesenchymal–epithelial transition factor; CSC, cancer stem cell; EMT, epithelial–mesenchymal transition; GPR49, G-protein coupled receptor 49; HCAM, homing cell adhesion molecule; HGFR, hepatocyte growth factor receptor; HNSCC, head and neck squamous cell carcinoma; Lgr5, leucine-rich repeat-containing G-protein coupled receptor 5; LSCC, laryngeal squamous cell carcinoma; NGFR, nerve growth factor receptor; NOD/SCID, non-obese diabetic/severe combined immunodeficient; Oct4, octamer-binding transcription factor 4; OSCC, oral squamous cell carcinoma; OTSCC, oral tongue squamous cell carcinoma; PCGF4, polycomb group RING finger protein 4; POU5F1, Pic-1, Oct1,2, Unc-86 transcription factor 1; PROM1, prominin-1; VM, vascular mimicry.
CSC markers in head and neck cancers
Fluorescence-activated cell sorting and magnetic bead sorting remain the most common methods to detect and isolate CSCs, wherein various cell markers were utilised, either individually or in combination. Besides their importance in understanding the complex behaviour of CSCs, these markers have emerged as valuable biomarkers and therapeutic targets in cancer. Here, we briefly outline recent findings of selected molecules that are more frequently applied as CSC markers in HNSCC.
CD44
The cluster differentiation CD44 is the key stem cell marker in solid tumours and the first used to study HNSCC-derived CSCs. CD44 is a multistructural and multifunctional transmembrane adhesion receptor that binds to several extracellular matrix (ECM) ligands, particularly to hyaluronic acid (HA; aka hyaluronan) – a glycosaminoglycan involved in pivotal tumourigenic events. CD44 has mediated cancer cell proliferation, migration, angiogenesis and stemness properties, ultimately leading to tumour progression and metastasis (Refs Reference Ludwig20–Reference Wang and Bourguignon23). Recently, Ludwig et al. (Ref. Reference Ludwig20) analysed the association of CD44 with the pro-angiogenic genotype in HNSCC using the Cancer Genome Atlas. Interestingly, they found that HNSCC has the second highest CD44 expression among all cancer types included in the Pan-Cancer Atlas. Moreover, using an orthotopic carcinogen-induced mouse model, CD44+ve expression was consistently upregulated at different stages of oral carcinogenesis, from dysplastic lesions to advanced carcinomas (Ref. Reference Ludwig20). The immunogenicity of CSCs was examined using primary human HNSCC samples and patient-derived xenografts. Surprisingly, CD44+ve CSCs in HNSCC revealed EMT features and were less immunogenic than other CD44−ve tumour cells when cultured with autologous CD8+ve tumour-infiltrating lymphocytes (TILs). Further, programmed death-ligand 1 (PD-L1) was selectively expressed on CD44+ve CSCs compared with CD44−ve cells (Ref. Reference Lee24). In addition, HNSCC-derived spheroids exhibited increased expression of CD44, whereas the levels of other putative CSC markers, such as CD24 and CD133, were not notably increased (Ref. Reference Byun25).
Human CD44 is encoded by the highly conserved CD44 gene on chromosome 11. Following extensive alternative splicing, it generates multiple variant isoforms, including the standard (CD44s) and variant (CD44v) forms, the latter representing a promising prognostic and therapeutic target in different cancers (Ref. Reference Wang26). In this regard, Wang et al. (Ref. Reference Wang26) showed that HNSCC cells (HSC-3) expressed at least four CD44 isoforms (v3, v6, v10) and CD44s. Of note, these CD44 isoforms mediated cancer cell migration, proliferation and cisplatin sensitivity. Importantly, tumours expressing the variant isoforms (v3, v6, v10) alone or in combination showed a greater proportion of metastatic lymph nodes and tumour progression than the standard form (Ref. Reference Wang26). A meta-analysis revealed a significant association between CD44 and worsening T stage, N status, higher tumour grades and 5-year overall survival (OS) rates in patients with HNSCC (Ref. Reference Chen27). However, despite its wide use in CSC studies, the increased levels of CD44+ve cells in some cohorts suggest that such population may not represent a ‘pure’ mass of CSCs. Thus, combining multiple surface markers, such as CD44 and aldehyde dehydrogenase (ALDH) has been increasingly used in HNSCC (Ref. Reference Kamarajan28).
ALDH
This family comprises a group of intracellular detoxifying enzymes that oxidise exogenous and endogenous aldehydes, hence mediating drug resistance in cancer patients (Ref. Reference Januchowski, Wojtowicz and Zabel29). ALDH has been considered a functional marker of HNSCC-derived CSCs (Refs Reference Clay11, Reference Kamarajan28, Reference Chen30). Among the first reports on this molecule in HNSCC, Chen et al. (Ref. Reference Chen30) showed that ALDH1+ve tumour cells displayed EMT features and radioresistance and represented a reservoir for tumour initiation. Unlike the copious expression of CD44, most HNSCC cells had low ALDH activity; nevertheless, ALDHhigh cells (1.0–7.8%) clearly co-expressed CD44 and sustained high tumourigenic potential in NOD/SCID mice (Ref. Reference Clay11). In agreement with these studies, HNSCC-derived ALDH1+ve CSCs had higher stemness traits, including sphere-forming capacity than ALDH1−ve cells (Ref. Reference Yu31).
These findings support the role of ALDH as a selective marker for HNSCC CSCs and hold promising therapeutic and prognostic utilities. In this regard, targeting ALDH with Aldi-6 (ALDH3A1 inhibitor) sensitised HNSCC cells to cisplatin and reduced tumour growth burden in vivo (Ref. Reference Kim32). Prince et al. (Ref. Reference Prince33) presented a feasible approach to prepare ALDHhigh CSC-based vaccines to induce anti-HNSCC immunity, implying a clinical utility to treat cancer patients. Recently, a multifactorial analysis revealed that HNSCC patients with negative immunoexpression of ALDH1A1 had 5.25 times higher OS than the ALDH1A1+ve group (P = 0.01). Furthermore, using univariate and multivariate analysis, only ALDH1A1 staining positivity showed a significant effect on OS in HNSCC patients compared with other CSC markers such as CD44 (Ref. Reference Szafarowski34). Of interest, HNSCC-ALDH1+ve CSCs were found to possess high levels of the transcriptional repressor Bmi-1, another putative marker of CSCs, regulating their stemness and drug resistance (Ref. Reference Chen30).
Bmi-1
The B-cell-specific Moloney murine leukaemia virus insertion site 1 (Bmi-1) is a key factor responsible for self-renewal and enrichment of stem cells. In their seminal work on characterizing CSCs in HNSCC, Prince et al. (Ref. Reference Prince10) reported a differential expression of Bmi-1 in the tumourigenic CD44+ve population, indicating a potential role for this molecule in tumour plasticity. Interestingly, Bmi-1 levels were abnormally upregulated in HNSCC patients, which correlated positively with chemo- and radioresistance (Ref. Reference Vormittag35). Thus, these findings have made it an attractive target for CSC examination in HNSCC studies. A recent comparative study found that Bmi-1 and BCL11B effectively discriminated between healthy and cancerous tissues in HNSCC patients, whereas ALDH1A1 and CD44 were both expressed to a comparable extent in these tissues (Ref. Reference Sharaf36). Interestingly, Bmi-1+ve cells were convincingly shown to be slow-cycled tumour-initiating CSCs that did not only initiated the tumour but also mediated cervical lymph node metastasis in a mouse model of chemically induced HNSCC (4-nitroquinoline-1-oxide: 4-NQO). Congruous with CSC features, Bmi-1+ve tumour cells were highly tumourigenic and chemo-resistant, whereas a combination of Bmi-1 inhibitor and cisplatin treatment effectively inhibited HNSCC (Ref. Reference Chen37).
Using a multicolour lineage tracing method in a 4-NQO-induced mouse model of OSCC, Tanaka et al. (Ref. Reference Tanaka38) reported that Bmi-1+ve cells could serve as oral CSCs. They showed that Bmi-1+ve cells were scattered in the developing tumours, which then proliferated to produce new patches – fuelling tumour growth and maintenance. However, some Bmi-1+ve cells remained single and gradually disappeared from the malignant tissue, implying that Bmi-1 was also expressed by differentiated cells, which have limited capacity to self-renew and maintain tumourigenesis (Ref. Reference Tanaka38). This finding also signifies the importance of employing multiple markers to better characterise CSCs. Of note, a recent study showed that Bmi-1 inhibition not only helped to abolish CSCs but also augmented PD1 blockade by activating tumour cell-intrinsic immunity, which hindered metastasis and prevented relapse in HNSCC (Ref. Reference Jia, Zhang and Wang39).
Other less frequently studied CSC markers are summarised in Table 1.
CSC plasticity: a partial phenotypic transition?
In addition to the aforementioned intratumoural heterogeneity patterns, a newer more nuanced model was recently proposed as ‘CSC plasticity’, whereby cells reversibly switch between stemness and differentiated states. Such transition is mediated by genetic and epigenetic alterations as well as by cues from key processes, particularly EMT and mesenchymal-to-epithelial transition (MET) (Refs Reference Fanelli, Naccarato and Scatena16, Reference Ghuwalewala40, Reference La Fleur, Johansson and Roberg41).
During embryogenesis, cells undergo highly dynamic and reversible shifts between epithelial and mesenchymal states. When the shift is towards the mesenchymal phenotype, cells undergo EMT and obtain potent migratory and invasive characteristics. EMT transcription factors include three main families: Snail, Twist and ZEB (Ref. Reference Yeung and Yang42). In contrast to EMT, cells may start losing these migratory features and shift towards an epithelial state by acquiring junctional attachments and apico-basal polarisation – a process referred to as MET (Ref. Reference Thiery43). Although the role of MET/EMT in cancer plasticity is still under investigation, it is nevertheless widely accepted that much of the intratumoural heterogeneity, invasion, metastasis and drug resistance are driven by these processes. CSCs can employ EMT to dissociate from the primary tumours, intravasate into the circulation and initiate new locoregional/distant colonies. Upon reaching a preferable metastatic niche, cells revert to an epithelial state (MET) to terminate migration, promote proliferation and seed new heterogeneous tumour colonies (Ref. Reference Nieto44).
In HNSCC, CSCs are often localised to the tumour invasive front, wherein both EMT and metastasis are executed, contrary to the upper layers of the tumour bulk, which remain largely epithelial (Ref. Reference Sterz45). Consistent with this, Chowdhury et al. (Ref. Reference Chowdhury46) showed that the tumour leading edges in a subset of OSCC were enriched by CD44high/ALDHhigh CSCs, which demonstrated greater proliferative and invasive activities. Notably, CD44high CSCs from HNSCC tumours revealed clear EMT features such as migration and invasion. Further, CD44high cells formed bilateral lung metastases in NOD-SCID mice, in contrast to CD44low, which failed to generate similar metastatic growths (Ref. Reference Davis47). When analysing EMT genes in 25 HNSCC cell lines, Johansson et al. (Ref. Reference La Fleur, Johansson and Roberg41) found that EMT-expressing cells were mainly CD44high with an enhanced motility. Of interest, these cells had low levels of epidermal growth factor receptor – a pattern associated with stemness (Refs Reference La Fleur, Johansson and Roberg41, Reference Johansson48). Moreover, the expression of Twist1, a key inducer of EMT, was correlated with radioresistance in HNSCC (Ref. Reference Johansson48). In support of these reports, Twist1 directly regulated the expression of the putative CSC marker Bmi-1. Furthermore, Twist1 and Bmi-1 were mutually essential to promote EMT and tumour-initiating capability and associated with unfavourable clinical outcomes in HNSCC (Ref. Reference Yang49). In OSCC, EMT characteristics such as ZEB1 overexpression and loss of E-cadherin were markedly higher in CD44high/CD24low CSCs, indicating that tumour cell stemness co-occurs with and is probably promoted by EMT (Ref. Reference Ghuwalewala40). Recently, 16 canonical EMT markers were surveyed in a pan-cancer cohort collected from various tumours, confirming the presence of EMT features in HNSCC patients (Ref. Reference Gibbons and Creighton50).
Recent evidence has shown that tumour cells do not necessarily undergo a complete phenotypic transition; rather, cells tend to execute partial (pEMT) or hybrid EMT by concurrently revealing epithelial and mesenchymal phenotypes (Ref. Reference Bakir51). Supporting the ‘CSC plasticity’ model, transcriptional profiles of ~6000 single cells from HNSCC patients showed that pEMT-expressing cancer cells were localised to the leading edge of primary tumours. These cells were in proximity to cancer-associated fibroblasts and their pEMT programme was deemed to be an independent predictor of nodal metastasis, tumour grade and adverse pathologic features (Ref. Reference Puram9). In this regard, it is logical to assume that CSCs utilise their mesenchymal gene repertoire at the tumoural front to invade and disseminate, while maintaining their epithelial characteristics to reseed and establish new metastatic growths. Such plasticity is influenced by many TME-related factors such as hypoxia (Ref. Reference Mimeault and Batra52).
Role of hypoxic microenvironment
Hypoxia, either persistent or temporary, is a feature of most solid tumours. Accumulating experimental data suggest hypoxia as a crucial inducer of EMT and CSC plasticity, wherein hypoxia-inducible factors (HIFs) are associated with tumour cell stemness, metastasis, angiogenesis and drug resistance (Refs Reference Mimeault and Batra52, Reference Lee and Simon53). Like other solid tumours, hypoxia ensues in HNSCC when the blood supply becomes insufficient due to tumour growth, vascular disturbances or metabolic stress. Besides its pro-stemness effect, hypoxia was shown to promote anti-apoptotic pathways and tumour aggressiveness, predict poor therapeutic response and induce the formation of functional invadopodia in HNSCC cells (Refs Reference Sasabe54–Reference Wiechec58). A recent study found that hypoxia-related genes were enriched in CD44+ve CSCs from patients with HNSCC. Interestingly, functional assays indicated that HIF-1α promoted stemness, drug resistance and EMT in these CD44+ve CSCs. It is noteworthy that inhibition of HIF1α-driven pathways reversed the CD44-mediated chemoresistance in vivo, implying new therapeutic opportunities in HNSCC (Ref. Reference Byun25). Although reports assessing the direct influence of hypoxia on CSCs in HNSCC are limited, hypoxia may mediate its pro-stemness effects through EMT. In this regard, hypoxia induced the expression of key EMT transcriptional factors and promoted pulmonary metastasis in OSCC (Refs Reference Wiechec58, Reference Huang59). Further, co-expression of hypoxia and EMT markers (Twist 2/Snip1 and HIF-1α, respectively) served as an independent prognosticator for both OS and disease-free survival in patients with tongue SCC (TSCC) (Ref. Reference Liang60). These reports suggest that hypoxia is not only a promoter of tumour stemness and EMT but can also regulate therapeutic response to anticancer agents. Additional evidence of the impact of hypoxia on cancer stemness can be found in reviews (Refs Reference Mimeault and Batra52, Reference Lee and Simon53, Reference Yeo61). One important aspect of hypoxia-induced stemness is the induction of an intriguing pattern of tumour vasculature, termed vascular mimicry (VM), which will be discussed in the next section.
Plasticity underlies tumour cell mimicry
Emerging evidence reveals that certain tumour cells can acquire intrinsic differentiation programmes of distinct cell types, including vascular, neuron and immune cell mimicry to survive harsh TME and facilitate tumour progression and clinical resistance.
Endothelial cell mimicry
Angiogenesis is a hallmark of cancer. However, the limited success of antiangiogenic strategies remains a daunting challenge in treating many solid tumours including HNSCC (Ref. Reference Ribatti62). In fact, despite the growing number of clinical trials, targeting the classical angiogenic pathways (e.g. vascular endothelial growth factor, VEGF) showed only a modest improvement in the OS of cancer patients. Unexpectedly, inhibiting the VEGF pathway has been associated with persistent tumour invasiveness, increased distant metastasis and worsening treatment outcome (Refs Reference Ebos63, Reference Pàez-Ribes64). Questions have therefore emerged regarding how tumour cells can survive such harsh hypoxic conditions and also how they can become more ‘metastable’ and resistant following treatment with angiogenic inhibitors.
Tumour vasculature has long been assumed to arise from pre-existing endothelial cells (ECs). However, a significant number of studies suggest that CSCs utilise their phenotypic plasticity to acquire differentiation programmes of distinct cell types, such as EC-like phenotype (Ref. Reference Seftor65). Indeed, the CSC marker CD44 has been implicated in promoting several tumour proangiogenic events (Ref. Reference Wang and Bourguignon23). In this regard, VM represents an alternative pattern of tumour microcirculation in which aggressive tumour cells mimic ECs by initiating perfusable networks of vessel-like structures in vitro (Refs Reference Salem and Salo66, Reference Hujanen67). Notably, tumour cells capable of VM share considerable molecular similarity with that of CSCs (Refs Reference Seftor65, Reference Salem and Salo66, Reference Fan68). For instance, up to 90% of CSCs were able to transdifferentiate into functional ECs in glioblastoma, the most common and aggressive brain tumour, implying that a significant portion of tumour vasculature has a neoplastic origin (Ref. Reference Ricci-Vitiani69). Recently, we showed that metastatic OSCC cells co-express the endothelial marker CD31 in vitro. Furthermore, tissue samples from OSCC patients revealed distinct CD31+ve mosaic VM lumens that also contained red blood cells (Refs Reference Hujanen21, Reference Almahmoudi70). Interestingly, and unlike VM-free regions, such VM-competent cells display CD44high/E-cadherinlow phenotype, denoting a common stemness-related state (Ref. Reference Hujanen21).
Apart from frank angiogenesis, tumour lymphatics play a pivotal role in cancer progression. In a recent study on basal-like breast cancer, CSCs were capable of undergoing lymphatic cell differentiation via the activation of the VEGF-C pathway by which lymphatic vessel-like channels were initiated. Further in vivo and in vitro experiments revealed that lymphangiogenic mimicry (LM) served as a conduit for CSCs to lymphatic vessels and accelerated lymphatic metastases (Ref. Reference Wang71). Our group has recently demonstrated that the lymphatic vessel endothelial hyaluronan receptor 1 (LYVE-1), a homologue of CSC marker CD44, is expressed by OSCC cells and promotes their dissemination and vessel-like formation (Ref. Reference Karinen72). Notably, patients with tumours expressing high levels of LYVE-1 tend to suffer more lymph node metastasis and have worse survival (Refs Reference Karinen72, Reference Karinen73). Of interest, LYVE-1 is a receptor for HA – a key component of ECM that influences, directly and indirectly, the self-renewal and maintenance of CSCs (Ref. Reference Chanmee74). Thus, targeting the LYVE-1 or VEGF-C pathway (or both) may represent a novel therapeutic approach to conquer CSC-driven lymphatic metastasis in HNSCC. Nevertheless, the potential clinical applications of LM remain unclear, warranting further in-depth investigations.
Immune and neuronal cell mimicry
In addition to the vascular cell phenotype, recent studies uncovered more intriguing differentiation programmes of CSCs, including the acquisition of immune and neuronal cell states to facilitate tumour progression (Refs Reference Gangoso75–Reference Zeng77). However, conventional bulk transcriptomic profiling can be ineffective in distinguishing such tumour-intrinsic programmes from other TME-infiltrating cells.
Based on scRNA-seq datasets and experimental models, an elevated immune-like transcriptional programme was recently seen in cells of multiple cancer types, which was progressively acquired during the course of malignant transformation. Specifically, an enrichment of B cell, T cell and myeloid cell signatures was revealed in tumour cells from lung, breast, kidney and pancreatic cancers. The score was, however, lower in tumour cells than in immune cells, implying a partial acquisition of this immune mimicry during progression from normalcy to neoplasm (Ref. Reference Gao76). Of note, such mimetic profile might adversely influence the prognostic value of immune cell signatures in cancer studies. Hence, through the exclusion of tumour cell-related immune genes, a new optimised immune response signature was proposed, which offered more reliable prognostic estimates (Ref. Reference Gao76). These findings are consistent with a recent work showing a striking gain in immune evasion capabilities of CSCs following the acquisition of a transcriptional module that hampered the immune response in glioblastoma multiforme (Ref. Reference Gangoso75). In primary HNSCC, Lee et al. (Ref. Reference Lee24) provided a mechanism by which long-lived CD44+ve CSCs can selectively evade host immune responses. Interestingly, CD44+ve cells expressed higher levels of PD-L1, which was associated with constitutive phosphorylation of signal transducer and activator of transcription 3 (STAT3) and decreased immunogenicity. Importantly, PD-1/PD-L1 blockade partially reversed the weakened immunogenicity and activated the TILs response against CD44+ve CSCs (Ref. Reference Lee24). Indeed, these data highlight the complex genetic and phenotypic changes of CSCs and their infinite ability to hijack the immune response and remodel TME. Although this is still a new area of research, it is paramount to investigate whether the immune mimicry is implicated in HNSCC, wherein mechanisms underpinning its immunosuppressive TME remain an enigma.
To survive and initiate metastatic colonisation in the brain, tumour cells must render their new neural niche to an amenable microenvironment. In this context, Neman et al. (Ref. Reference Neman78) showed that HER2+ve, breast-to-brain metastatic (BBM) CSCs can attain neuronal phenotype by expressing and metabolizing gamma-aminobutyric acid (GABA) – the chief inhibitory neurotransmitter in mammalian brains. GABA was utilised by tumour cells to promote their proliferation and GABAergic neuronal properties. Further, BBM cells expressed Reelin, a critical neuronal glycoprotein for brain development, which was directly associated with HER2 (Ref. Reference Neman78). Supporting these observations, a recent preprint (not peer-reviewed) study showed that small-cell lung cancer (SCLC) cells undergo neuronal mimicry – a transition towards neuronal phenotype during tumour progression and metastasis. More importantly, this neuronal mimicry was critical for establishing SCLC growth in the brain, whereby tumour cells secrete neuronal pro-survival factors (e.g. Reelin) to recruit astrocytes and promote brain metastases (Ref. Reference Fangfei79). In essence, although brain metastasis in HNSCC is a rare event, comprising <1% of all cases (Ref. Reference Barrett80), these reports further assert the concept of CSCs and stress the need for more studies in this exciting area with much more to reveal.
Oral microbiota and CSCs: emerging evidence
Recently, the ‘polymorphic microbiome’ has been proposed as a new hallmark of cancer (Ref. Reference Hanahan81). Dysbiosis of the commensal microbiota can contribute, both positively and negatively, to the initiation and progression of HNSCC and, through immunomodulation, to anticancer drug response (Ref. Reference Metsäniitty5). Recent research has advanced our understanding of the interactions between microbiota and tumour cells, and there is a growing appreciation of the potential of microbiota to transform treatment strategies for cancer patients. This review briefly outlines a few examples of microbiota commonly implicated in HNSCC, to then focus on their possible role in tumour plasticity.
Oral cavity hosts diverse microbial communities inhabiting different sites such as teeth, saliva, tongue and other mucosal surfaces. Using germ-free animal models, particular microorganisms, chiefly but not exclusively bacteria, were shown to impact key features associated with tumour cell stemness such as EMT, proliferation, migration and invasion. Among these, two prominent oral bacteria, Porphyromonas gingivalis (P. gingivalis) and Fusobacterium nucleatum (F. nucleatum), influenced oral carcinogenesis and tumour progression. For example, infection with P. gingivalis, a major anaerobic Gram-negative periodontopathogen, induced EMT-driven morphological changes in OSCC cells and augmented their migratory and invasive capacities. Importantly, P. gingivalis-infected tumour cells exhibited evident plasticity features, including the upregulation of CSC markers (CD44 and CD133) and chemotherapeutic resistance (Ref. Reference Ha82). Using a murine model of periodontitis-associated oral TSCC, infection with both P. gingivalis and F. nucleatum markedly enhanced the tumour size and invasiveness. Furthermore, administration of these periodontopathogens significantly induced the activation of STAT3 – an important regulator of CSCs in HNSCC (Ref. Reference Binder Gallimidi83). Exposure to lipopolysaccharides from P. gingivalis, F. nucleatum or Escherichia coli resulted in a strong upregulation of certain EMT transcripts (vimentin, Snail, Twist) in OSCC cells. On the other hand, the epithelial adhesion molecule E-cadherin was downregulated, implying shifting towards a mesenchymal state. Notably, these infected tumour cells showed morphological changes resembling those of fibroblasts, while unstimulated cells maintained a classical epithelial cobble-stone morphology (Ref. Reference Abdulkareem84).
Epstein–Barr virus (EBV) is a human herpesvirus associated with several malignancies such as nasopharyngeal carcinoma (NPC). Lun et al. (Ref. Reference Lun85) found that CSC markers (CD44 and SOX2) were overexpressed in a minor population of EBV+ve NPC cells, which were resistant to chemotherapy and showed high spheroid formation efficiency. Consistent with CSC properties, the authors revealed that the sorted CD44+ve cells generated a heterogeneous population of both CD44+ve and CD44−ve cells (Ref. Reference Lun85). The exact mechanisms by which EBV and other pathogenic microbiota induce tumour cell stemness are still being elucidated. For instance, the latent membrane protein 1 (LMP1) constitutes a key oncoprotein of EBV. Interestingly, Kondo et al. (Ref. Reference Kondo86) revealed that EBV induces the development of CD44high/CD24low phenotype of CSCs and cancer progenitor cells in NPC via LMP1. Moreover, LMP1 induction in nasopharyngeal epithelial cells resulted in high tumourigenicity, rapid proliferation and enhanced self-renewal abilities. Morphologically, LMP1-expressing cells changed into fibroblast-like, spindle-shaped cells (Ref. Reference Kondo86).
HPV-induced oropharyngeal HNSCC is one of the most rapidly increasing cancers in high-income countries, also affecting younger individuals (Ref. Reference Lechner87). Despite the evident prognostic value of HPV status in HNSCC patients, its influence on CSCs and cell stemness remains poorly understood and sometimes conflicting. For instance, HPV status was shown to not correlate with the proportion of ALDHhigh CSCs in HNSCC (Ref. Reference Tang88). By contrast, another study showed that HPV16+ve HNSCC had a 62.5-fold greater intrinsic CSC pool than HPV−ve cells (Ref. Reference Zhang89). Further, transfecting tumour cells with HPV16 genome enhanced CSC features including ALDH activity, tumour growth, migration/invasion and self-renewal capacity (Ref. Reference Lee90). However, it is perhaps difficult to explain how HPV+ve tumours – characterised by substantially better prognosis – harbour higher CSCs, which render the tumours more aggressive and drug-resistant. Nevertheless, recent studies have suggested that HPV could impact the CSC population in HNSCC after initiating the therapeutic regimen. In this sense, Reid et al. (Ref. Reference Reid91) demonstrated that HPV−ve tumours had significant elevations in CD44+ve/ALDH+veCSCs following irradiation, with greater escalation than HPV+ve cell lines.
Collectively, although these illustrative examples are rather limited and comprise small sample sizes, they encourage further research to uncover the relationship between oral microbiota and CSCs and how it might influence carcinogenesis in head and neck tissues.
A need for new models?
The characteristics of CSCs are traditionally assessed in vitro using numerous three-dimensional (3D) techniques (comprehensively reviewed in references Reference Reid91, Reference Langhans92). Among these, the sphere (or spheroid-like) formation assay is commonly used to assess the ability of CSCs to grow in extremely low attachment/serum conditions and form tumourspheres. These tumourspheres are also termed ‘orospheres’ when formed by HNSCC CSCs, indicating their origin from the oral cavity and head and neck tumours (Ref. Reference Krishnamurthy and Nör94). Typically, tumourspheres are generated using scaffold-free (e.g. hanging drop cultures and low adhesion plates) and scaffold-based models (Fig. 2a and b). The latter scaffold-based 3D cultures provide more physiologically relevant conditions, particularly when incorporating biologically derived hydrogels such as collagen, fibrin or Matrigel. These matrices facilitate cell-to-cell and cell-to-ECM interactions, thereby allowing more reliable assessment of CSC tumourigenicity, stemness and drug response, including possible chemo-/radioresistance. In particular, Matrigel, a matrix extracted from Engelbreth–Holm–Swarm (EHS) mouse sarcoma tissue, has been broadly used to assess CSC features due to its high content of ECM proteins, growth factors and proteoglycans (Refs Reference Langhans92–Reference Aramini95). However, animal-derived matrices, such as Matrigel, have several limitations, making them suboptimal for human CSC research, including non-human origin, inter-batch variations and presence of several undefined factors (Ref. Reference Pamarthy and Sabaawy96). Therefore, the safety and efficacy of 85% of the drugs identified in such models are not translated to early clinical trials (Ref. Reference Ledford97).

Fig. 2. Examples of models used to assess the features of cancer stem cells (CSCs). Ex vivo models are broadly categorised as follows: (a) scaffold-free cultures, such as hanging drops, low-attachment plates and suspension cultures, to assess the formation of tumourspheres, and (b) scaffold-based models, including the use of biological hydrogels such as Matrigel and Myogel, for three-dimensional assays. (c) In vivo models are mainly used to assess the capacity of CSC-like cells to initiate and sustain new growths (and hence, they are also termed tumour-initiating/propagating cells). The most commonly used models include non-obese diabetic/severe combined immunodeficient (NOD/SCID) and NOD/SCID IL2Rγ null mice. (d) Humanised animal models (e.g. XactMice and GSS zebrafish) represent promising potential platforms to assess the human CSC hypothesis in a more relevant niche encompassing human hematopoietic stem and progenitor cells (HSPCs), which are able to recreate the original tumour microenvironment observed clinically. HSPCs are expanded ex vivo and injected into the NOD/SCID gamma mice. (e) In addition, zebrafish larvae may represent an attractive and cost-effective model in CSC research, particularly for assessing tumour progression and drug resistance.
To provide a reliable alternative to animal-derived matrices, our group developed ‘Myogel’, a matrix isolated from human uterus leiomyoma that provides a physiologically relevant 3D milieu for ex vivo cancer modelling. Although its proteome is substantially different from murine EHS-derived matrices, Myogel comprises key ECM proteins such as laminin, collagen (types IV, XII and XIV), tenascin-C, heparan sulphate proteoglycans, nidogen and EGF (Ref. Reference Salo98). A balanced physiological pH environment is important for the regulation and maintenance of stem cell activities including proliferation, viability and differentiation (Ref. Reference Teo, Mantalaris and Lim99). In this context, Myogel pH is neutral and more stable during cell culture than that of Matrigel, hence providing a good control of CSC experiments. Importantly, key CSC-related properties were more efficiently represented in Myogel, including tumour cell migration, invasion and response to targeted anti-HNSCC therapy (Refs Reference Salo98, Reference Naakka100, Reference Tuomainen101). Furthermore, primary HNSCC cell lines showed a greater tendency to form VM in Myogel, whereas human ECs formed consistent and dense tubes throughout the matrix, suggesting that ECM is an important modulator of the tumour cell-derived tubulogenesis (Ref. Reference Hujanen21). Thus, when selecting a 3D scaffold for CSC studies, it is important to consider matrices that sustain stemness traits and closely recapitulate the structural and molecular features of human TME.
Tumour-initiating/propagating capacity is considered to be the gold standard for identifying CSCs. Currently, most in vivo studies of CSCs rely upon tumour engraftment into severely immunocompromised mouse models – typically NOD/SCID and NOD/SCID IL2Rγ null mice (Fig. 2c). These mice are B cell- and T cell-deficient models and exhibit defective activity of dendritic cells, macrophages and natural killer cells, thus enhancing the chances of successful xenotransplantation (Ref. Reference Skidan and Steiniger102). While they led to most seminal findings on CSCs, these models do not reliably represent the native tumour stroma, including the dearth of cytokines regulating CSC activities. Thus, for more rigorous assessment of the human CSC hypothesis, tumour cells need to be transferred into mice installed with all the requisite human supporting cells (Ref. Reference Kelly103). To overcome such constraints, Morton et al. (Ref. Reference Morton104) developed a humanised xenochimeric mouse model of HNSCC (XactMice) comprising human hematopoietic stem and progenitor cells (HSPCs) able to recreate the original TME observed clinically. Following the harvesting of HSPCs from either donated cord blood or adult peripheral blood, cells were expanded ex vivo and injected into sub-lethally irradiated NOD/SCID gamma mice to generate XactMice, wherein HNSCC cells were later engrafted (Fig. 2d). Interestingly, XactMice tumours showed epithelial, stromal and immune gene signature that aligns more closely to the original TME observed clinically – an effect partly mediated by HSPC-/tumour cell-driven cytokines (Ref. Reference Morton104). Additionally, the same group developed a dual infusion protocol of HSPCs and mesenchymal stem cells, resulting in higher degrees of ‘humanisation’ of the HNSCC mouse model. This includes incremental human bone marrow engraftment, excessive increase in human immune cells and intratumoural homing with better lineage reconstitution. This optimised model more closely resembles that of the originating patient's tumour, suggesting an enhanced capability to accurately recapitulate a human TME (Ref. Reference Morton105).
Another platform that could be useful for CSC research is Zebrafish – a model that has been increasingly used in anticancer screening. Recently, our group utilised zebrafish larvae as a xenograft model of human OSCC to evaluate tumour cell metastasis, VM formation and personalised drug response (Fig. 2e) (Refs Reference Hujanen21, Reference Karinen72, Reference Wahab106, Reference Al-Samadi107). Indeed, zebrafish has several advantages over murine models with regard to its efficiency, feasibility and cost- and labour-effectiveness. Further, zebrafish larvae are optically transparent, making them an excellent candidate for live imaging, which can be particularly advantageous in CSC research (Refs Reference Hujanen21, Reference Karinen72). However, similar to mice, the zebrafish model is devoid of critical cytokines, chemokines and other TME factors released in human patients. To alleviate this limitation, Rajan et al. (Ref. Reference Rajan108) pioneered the first humanised triple GSS (GM-CSF, SCF, SDF1α) transgenic zebrafish that express multiple human hematopoietic-specific cytokines. By transplanting primary HSPCs and leukaemia cells, they showed that their model exhibits hematopoietic niche homing that more precisely represents the behaviour of human leukaemia. Further, this humanised model promoted survival, self-renewal and multi-lineage differentiation of HSPCs (Ref. Reference Rajan108). Although this platform, to our knowledge, has not yet been harnessed to study head and neck tumourigenesis and CSCs, the findings pave the way for a new modelling paradigm in humanised animal-based research.
Taken together, developing physiologically relevant ex vivo and in vivo models that faithfully recapitulate CSCs' niche and enable their characterisation and therapeutic targeting will facilitate the translation of basic discoveries to clinical practice in a timely manner. Such approaches are paramount for the development of novel drugs that can selectively target and destroy CSCs.
Conclusions, knowledge gaps and future perspectives
HNSCC encompass highly heterogeneous tumours, wherein CSCs seem to play multifaceted roles with regard to tumour progression, metastasis, clinical resistance and possible relapse. Although the CSC model has generated considerable controversy, recent technological advances, such as scRNA-seq, have led to unprecedented understanding of CSCs and their intricate interplay with other TME factors. However, much work remains to be done before this understanding can be translated into successful anticancer therapies, with the prognosis for HNSCC patients generally remaining dismal. To this end, several areas need to be elucidated, including, among others, pathways involved in CSC signalling, considering in particular oral microbiota, the molecular underpinning of CSC-driven drug resistance and immune cell mimicry. Another challenge is to develop more relevant models for CSC research. Despite the tremendous progress made with humanised animal platforms, they remain relatively immature with several shortcomings. For instance, besides cost and labour intensiveness, development of xenogeneic graft-versus-host disease (GVHD) is a major obstacle in such models. GVHD not only affects the number of viable experimental animals but also complicates data interpretation by overlaying a second set of diseases (Ref. Reference Greenblatt109). Nevertheless, these emerging alternative systems, including humanised zebrafish, remain a promising approach with a high potential for improvement to better identify CSCs and to catalogue the diverse cellular activities in HNSCC.
Lastly, but importantly, there is a pressing need to identify specific (i.e. exclusive) markers for CSCs in HNSCC. To our knowledge, there are no available universal markers that can label CSCs alone to date. On the contrary, several current markers are also expressed by other cell types, such as normal stem cells. In particular, the recognition of pEMT paradigm has made it more technically challenging to trace CSCs with such dynamic ‘identity-switching’ capacity. Thus, combining different putative labels of CSCs is recommended. Importantly, novel therapies need to be carefully designed to exclusively target this subpopulation of tumour cells without abrogating tissue stem cells or disrupting vital functions (Refs Reference Prince33, Reference Skidan and Steiniger102). In the therapeutic sense, anti-cancer vaccines targeting CSCs through dendritic cells and other immune cell types have shown promising results in HNSCC and could be further elaborated in the future (Ref. Reference Prince33). In conclusion, tumour cell plasticity and CSCs represent an exciting and expanding battleground in cancer research, with great implications for cancer therapy that are only beginning to be appreciated in head and neck oncology.
Acknowledgements
The authors gratefully acknowledge the funders of this study: The Jane and Aatos Erkko Foundation, the Finnish Dental Association (Apollonia) and the Cancer Society of Finland. Figures were created with BioRender.com.
Author contributions
A. S. researched data and wrote the article. T. S. reviewed the manuscript before submission.
Conflict of interest
None.