Introduction
Situated on the current onshore margin of Victoria Land, East Antarctica (c. 161°E, 78°S), is a remarkable deglaciated landscape of channels up to 30 km long, potholes and plunge pools tens of metres deep and up to 70 m across, and areas of stripped and corrugated bedrock ranging in size from 100 m2 to 3 km2 (Fig. 1) that provides convincing evidence for the existence of significant subglacial water flows beneath the Antarctic ice sheet (Sugden et al. Reference Sugden, Denton and Marchant1991, Reference Sugden, Bentley and Ó Cofaigh2006, Sugden & Denton Reference Sugden and Denton2004, Denton & Sugden Reference Denton and Sugden2005, Lewis et al. Reference Lewis, Marchant, Kowalewski, Baldwin and Webb2006, Marchant et al. Reference Marchant, Jamieson and Sugden2011). The size of the constituent landforms is comparable with the famous ‘Channelled Scablands’ landscape of the north-western USA, whose formation has been attributed to rapid flood events resulting from periodic drainage of ice-dammed glacial Lake Missoula during decay of the Pleistocene Laurentide Ice Sheet (Baker & Bunker Reference Baker and Bunker1985). However, the routing of the channels in Victoria Land does not appear to be controlled by simple topographical gradients; rather it is clearly subglacial in origin, as modelled from classical Shreve theory (Shreve Reference Shreve1972, Marchant et al. Reference Marchant, Jamieson and Sugden2011; see Fig. 2 for conceptual diagram). The downstream edges of channel networks are characterized by mega-ripples comprised of cobble-sized boulders, probably formed by the subglacial floodwaters. Dating of these deposits across the region indicates that they are at least 14 m.y.a. (Sugden & Denton Reference Sugden and Denton2004, Margerison et al. Reference Margerison, Phillips, Stuart and Sugden2005); hence, this channelized landscape was carved into the subglacial surface at a time when the East Antarctic Ice Sheet extended well beyond current limits. This labyrinthine landscape of subglacially carved channels stands as a clear monument to the intensity of subglacial water flows beneath the Antarctic ice sheet.

Fig. 1 The ‘Labyrinth’ subglacially-formed, and now deglaciated, channel system in western Wright Valley, Dry Valleys region, East Antarctica. Aerial view to the east. Channels are cut into dolerite. Individual channels are up to 100 m deep. The North Fork of Wright Valley is in the upper left background. Some potholes on the interfluves are littered with dolerite blocks, some of which are imbricated (photograph courtesy David Sugden; caption modified from Denton & Sugden Reference Denton and Sugden2005).
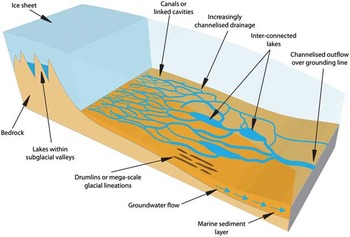
Fig. 2 Conceptual diagram of the typical situation of subglacial lakes and configuration of water flows beneath the Antarctic ice sheet.
Therefore, it is perhaps surprising that, until the last decade, water flows beneath the Antarctic ice sheet have received little attention in the scientific literature. However, over the last decade several developments have transformed our understanding of subglacial water flows beneath the Antarctic ice sheet. These include: i) a growing realization of the significance of subglacial water flows to continental-scale ice dynamics (e.g. Siegert & Bamber Reference Siegert and Bamber2000, Pattyn et al. Reference Pattyn, De Smedt and Souchez2004, Fricker & Scambos Reference Fricker and Scambos2009), oceanic circulation (e.g. Evatt et al. Reference Evatt, Fowler, Clark and Hulton2006, Payne et al. Reference Payne, Holland, Shepherd, Rutt, Jenkins and Joughin2007) and nutrient productivity (e.g. Statham et al. Reference Statham, Skidmore and Tranter2008, Skidmore Reference Skidmore2011), ii) the development of remote sensing techniques that facilitate recovery or inference of subglacial conditions beneath the contemporary ice sheet (e.g. Robin et al. Reference Robin, Drewry and Meldrum1977, Blankenship et al. Reference Blankenship, Bentley, Rooney and Alley1986, Smith Reference Smith1997a), iii) enhanced imaging of subglacial hydraulic networks across continental shelf regions previously covered by the expanded ice sheet (e.g. Nitsche et al. Reference Nitsche, Gohl, Larter, Hillenbrand, Kuhn, Smith, Jacobs, Anderson and Jakobsson2013), and iv) the constant refinement of glaciohydrological theories from other field sites, notably in Greenland (e.g. Zwally et al. Reference Zwally, Abdalati, Herring, Larson, Saba and Steffen2002, Tedstone et al. Reference Tedstone, Nienow, Sole, Mair, Cowton, Bartholomew and King2013).
The aim of this paper is to provide a brief overview of the current state of knowledge of subglacial hydrology across Antarctica, beginning by placing into context how knowledge of subglacial hydrology has grown from earlier studies mostly of alpine glaciers but more recently of those in the Arctic. This is followed by a discussion of where most hydrological attention in Antarctica has been focused until recently, namely the identification and exploration of subglacial lakes, before moving on to discuss examples of our burgeoning understanding of dynamic flows of water beneath the ice sheet. While many recent advances in understanding have been made, there remains much to be understood about the ways in which water is generated, stored, flows beneath and leaves the Antarctic ice sheet, and we therefore attempt to identify some of the grand challenges for improving our understanding of Antarctic subglacial hydrology. Figure 3 is provided as a reference guide for the Antarctic features and locations discussed.

Fig. 3 Antarctic locations referred to in the text, using the ice-surface velocity map of Rignot et al. (Reference Rignot, Mouginot and Scheuchl2011), superimposed over the MODIS Mosaic of Antarctica as a basemap. The locations of subglacial lakes are denoted by circles, and the subglacial-flood landforms shown in Fig. 1 are located by the black star. An inventory of all known subglacial lake locations in Antarctica is provided by Wright & Siegert (Reference Wright and Siegert2012). AB=Aurora Basin, AL=Adventure Lakes, BG=Byrd Glacier, BIS=Bindschadler Ice Stream, DA=Dome A, DC=Dome C, DF=Dome F, EAIS=East Antarctic Ice Sheet, EIR=Engelhardt Ice Ridge, EIS=Evans Ice Stream, GSM=Gamburtsev Subglacial Mountains, IIS=Institute Ice Stream, KIS=Kamb Ice Stream, LD=Law Dome, MAIS=MacAyeal Ice Stream, MIS=Mercer Ice Stream, PIG=Pine Island Glacier, RBIS=Roi Baudouin Ice Shelf, RG=Ragnhild Glaciers, RIR=Raymond Ice Ridge, RIS=Rutford Ice Stream, RL=Recovery Lakes, SC=Soya Coast, SD=Siple Dome, SG=Slessor Glacier, SLE=Subglacial Lake Ellsworth, SLV=Subglacial Lake Vostok, SLW=Subglacial Lake Whillans, TD=Taylor Dome, ThwG=Thwaites Glacier, TotG=Totten Glacier, VL=Victoria Land, WAIS=West Antarctic Ice Sheet, WB=Wilkes Basin, WD=WAIS divide, WIS=Whillans Ice Stream, WL=Wilkes Land.
Context
Scientists have been studying glacier hydrology for well over 40 years (for detailed reviews see Hubbard & Nienow Reference Hubbard and Nienow1997, Fountain & Walder Reference Fountain and Walder1998, Cuffey & Paterson Reference Cuffey and Paterson2010). Important concepts from these studies include: i) glacial meltwater can be generated supraglacially, englacially and subglacially from various sources, ii) meltwater follows different flowpaths through glaciers (Cuffey & Paterson Reference Cuffey and Paterson2010), iii) flowpaths can evolve in form with consequent impacts on the efficiency of water routing (Shreve Reference Shreve1972, Nienow et al. Reference Nienow, Sharp and Willis1998), and iv) the form and efficiency of the subglacial drainage system exerts a significant impact on the motion of the overlying ice flow (Iken & Bindschadler Reference Iken and Bindschadler1986, Mair et al. Reference Mair, Nienow, Sharp, Wohlleben and Willis2002). These concepts all hold for temperate glaciers, through which water flow is unrestricted by thermal considerations. For many years, the study of water flow through polythermal glaciers remained relatively unpursued, due in part to the conventional assumption that large proportions of cold ice would act to limit significant water flows, thereby preventing significant subglacial drainage system evolution and any hydraulic instabilities that might exert significant impacts on ice motion. Work by Müller & Iken (Reference Müller and Iken1973) on White Glacier, Arctic Canada, contradicted this assumption at an early stage, but it was not until a series of field studies on Arctic polythermal glaciers during the 1980s and 1990s that it became more readily accepted that drainage systems at polythermal glaciers do evolve, and that this can have readily observable impacts on patterns of ice flow (e.g. Hock & Hooke Reference Hock and Hooke1993, Bingham et al. Reference Bingham, Hubbard, Nienow and Sharp2008). Translation and upscaling of these concepts to various locations on the Greenland ice sheet has brought the issue to global attention (Zwally et al. Reference Zwally, Abdalati, Herring, Larson, Saba and Steffen2002, Van de Wal et al. Reference Van de Wal, Boot, van den Broeke, Smeets, Reijmer, Donker and Oerlemans2008, Bartholomew et al. Reference Bartholomew, Nienow, Mair, Hubbard, King and Sole2010, Sundal et al. Reference Sundal, Shepherd, Nienow, Hanna, Palmer and Huybrechts2011, Tedstone et al. Reference Tedstone, Nienow, Sole, Mair, Cowton, Bartholomew and King2013), and shown the significance of hydrological processes for ice dynamics at the ice-sheet-scale (e.g. Price et al. Reference Price, Payne, Catania and Neumann2008, Shannon et al. Reference Shannon, Payne and Bartholomew2013).
Antarctica continues to present a distinct glaciohydrological context. Under current climatic conditions the 0°C isotherm is rarely reached anywhere across West or East Antarctica, hence surface melt is limited to non-existent, and supraglacially-forced processes, which are considered to be responsible for inducing ice dynamic feedbacks in Arctic polythermal glaciers, cannot occur. A caveat to this is presented by the warmer, northward-trending Antarctic Peninsula region, in which it is conceivable that supraglacially-forced englacial connections could be established, thereby inducing polythermal-glacier drainage-system evolution and dynamic effects similar to those observed in the Arctic. Although this process has not been observed directly on Antarctic Peninsula polythermal glaciers, indirect evidence for its feasibility is provided by the demonstrable establishment of supraglacial-subglacial water connections on fringing ice shelves, which occasionally significantly impacts ice shelf stability and the glaciers formerly buttressed by them (Glasser & Scambos Reference Glasser and Scambos2008). However, for the Antarctic Peninsula glaciers overall, even though supraglacial-subglacial connections might occur, it is probable that their significance in any form of ice dynamic response mediation (cf. Hulbe et al. Reference Hulbe, Scambos, Youngberg and Lamb2008) is far outweighed by their collective and dramatic response to oceanic forcing along the coastline (Pritchard et al. Reference Pritchard, Arthern, Vaughan and Edwards2009).
This paper will not discuss the Antarctic Peninsula region further and will focus on the West and East Antarctic ice sheets, which comprise the vast majority of ice cover across the continent, and for which the subglacial hydrology remains only tentatively understood. A conceptual diagram for the following discussion is provided in Fig. 2.
Subglacial lakes
Beneath the contemporary continental ice sheets of Antarctica the identification and interrogation of subglacial hydrological phenomena is dependent upon multiple remote sensing techniques. In the late 1960s, scientists using airborne radar to survey ice thickness across the continent reported very clear, unusually strong and flat basal reflectors at many localities, which they interpreted as distinct bodies of collected subglacial water, or ‘subglacial lakes’ (Robin et al. Reference Robin, Drewry and Meldrum1977). Subglacial lakes are found wherever sufficient water is generated at the base of the ice sheet and gathers into depressions in hydraulic potential (Dowdeswell & Siegert Reference Dowdeswell and Siegert2003) and where inflow at least equals, if not exceeds, outflow. For several decades, the study of Antarctic subglacial hydrology has been synonymous with the identification of subglacial lakes, and elucidation of their formation and behaviour. This is because they are clearly identified in radio echo sounding (RES) data (e.g. Robin et al. Reference Robin, Drewry and Meldrum1977, and Fig. 4) and their significance is manifold, for example in their capacity to support life or impact on overlying ice dynamics (e.g. Siegert et al. Reference Siegert, Kennicutt II and Bindschadler2011). Successive inventories of Antarctic subglacial lakes have been published; initially using only the clearest lake-like returns visible in RES data but progressively incorporating ancillary forms of satellite observations (e.g. Fricker et al. Reference Fricker, Scambos, Bindschadler and Padman2007, Wright & Siegert Reference Wright and Siegert2012) and multiple-attribute processing of new digital radar data (e.g. Carter et al. Reference Carter, Blankenship, Peters, Young, Holt and Morse2007). The most recent inventory provides the locations of 379 subglacial lakes (Wright & Siegert Reference Wright and Siegert2012). The largest lakes are clearly expressed by depressions in the ice surface (e.g. Ridley et al. Reference Ridley, Cudlip and Laxon1993), which has been mapped from satellite observations across the continent (Bamber et al. Reference Bamber, Gomez-Dans and Griggs2009). It is therefore probable that all of the most substantial subglacial lakes across Antarctica have now been identified. However, even the basic dimensions of most of the lakes are not known, i.e. depth and volume, and there are undoubtedly many smaller lakes beneath the ice cover, which will continue to be discovered as RES surveys continue to explore the subglacial environment (Wright & Siegert Reference Wright and Siegert2012). Recent work by Livingstone et al. (Reference Livingstone, Clark, Woodward and Kingslake2013) offers potential in efforts to pinpoint the locations of smaller lakes where knowledge of the bed is good.

Fig. 4 Radargrams of the West Antarctic subglacial hydrological environment. a. Subglacial highlands near the West Antarctic divide showing steep, complex topography and well-preserved internal layers; note the bright reflection 27 km along-track indicating pooled water. b. The margin of Evans Ice Stream demonstrating the contrast between the ice stream, characterized by a bright reflection and absent-layering (left) and dimmer reflection and well-preserved layering in the slow-moving area (right). c. Flat and bright reflectors at the base of subglacial valleys; note their non-specular nature indicating the presence of saturated sediment, rather than a ‘definite lake’ (cf. Carter et al. Reference Carter, Blankenship, Peters, Young, Holt and Morse2007).
Before the last decade, most research on subglacial lakes explicitly or implicitly treated them as isolated, relatively stable, ‘closed-system’ phenomena. This led many to conceive of subglacial lakes as potential refugia, isolated and unique from elsewhere on Earth for millions of years (e.g. Priscu et al. Reference Priscu, Adams, Lyons, Voytek, Mogk, Brown, McKay, Takacs, Welch, Wolf, Kirshtein and Avci1999). This arresting notion motivated several high profile efforts to sample subglacial lake environments directly over recent years. Direct sampling was also driven by the potential of the lake bottom sediment profiles to record the overlying ice history (Bentley et al. Reference Bentley, Christoffersen, Hodgson, Smith, Tulaczyk and Le Brocq2011). Scientific drilling projects at the subglacial lake sites of Vostok and Ellsworth were particularly motivated by the search for subglacial life. Lake Vostok, East Antarctica, is the continent’s largest known subglacial lake with an estimated volume of 5400 km3 (Studinger et al. Reference Studinger, Bell and Tikku2004). It contains water that actively circulates and accretes onto the underside of the ice sheet at its southern end (Siegert et al. Reference Siegert, Kwok, Mayer and Hubbard2000, Bell et al. Reference Bell, Studinger, Tikku, Clarke, Gutner and Meertens2002), and preliminary samples of the accreted ice have provided evidence for the presence of microorganisms in the lake (Shtarkman et al. Reference Shtarkman, Kocer, Edgar, Veerapaneni, D’elia, Morris and Rogers2013). Lake Ellsworth fills an overdeepened former fjord flanking the Ellsworth Mountains in West Antarctica, which contains 1.37 km3 of water (Woodward et al. Reference Woodward, Smith, Ross, Thoma, Corr, King, King, Grosfeld, Tranter and Siegert2010). During 2012–13, another subglacial lake, Lake Whillans, West Antarctica, was the subject of scientific drilling investigations. Lake Whillans covers a c. 60 km2 region beneath Whillans Ice Stream and is a much shallower feature, probably only c. 2 m deep across much of its extent (Christianson et al. Reference Christianson, Jacobel, Horgan, Anandakrishnan and Alley2012, Horgan et al. Reference Horgan, Anandakrishnan, Jacobel, Christianson, Alley, Heeszel, Picotti and Walter2012). Although Lake Whillans may harbour life and access to the subglacial sediments may provide information on the ice-flow history (Fricker et al. Reference Fricker, Powell and Priscu2011), the initial discovery in 2007 by satellite altimetry, exposing it as a site of distinct cyclical ice-surface rise and fall (Fricker et al. Reference Fricker, Scambos, Bindschadler and Padman2007), pointed to strong dynamism in the subglacial hydraulic environment, motivating further investigation.
Over the last decade, a significant body of evidence has accumulated to demonstrate that many subglacial lakes form part of an interconnected network, draining up-gradient locations to down-gradient locations and ultimately to the ice sheet margin. This hypothesis was established by Dowdeswell & Siegert (Reference Dowdeswell and Siegert2003), but the first observational confirmation of its veracity was provided by Spikes et al. (Reference Spikes, Csatho, Hamilton and Whillans2003) and Gray et al. (Reference Gray, Joughin, Tulaczyk, Spikes, Bindschadler and Jezek2005), who interpreted satellite signals of isolated ice surface elevation change in the Siple Coast region of West Antarctica as the expressions of episodic drainage/fill events in connected subglacial lakes. Subsequently, Wingham et al. (Reference Wingham, Siegert, Shepherd and Muir2006) used radar altimetry to record movement between pairs of subglacial lakes over tens of km in East Antarctica. Fricker et al. (Reference Fricker, Scambos, Bindschadler and Padman2007) and Fricker & Scambos (Reference Fricker and Scambos2009) utilized satellite laser altimetry to record pervasive, connected subglacial lake activity across lower Mercer and Whillans ice streams in West Antarctica. Smith et al. (Reference Smith, Fricker, Joughin and Tulaczyk2009) collated all satellite laser altimeter observations of vertical ice surface change across Antarctica between 2003 and 2008 that could conceivably reflect subglacial lake drainage/filling events to produce an inventory of 124 ‘active’ subglacial lakes across the Antarctic ice sheet. These observations strongly suggest that the treatment of subglacial lakes as isolated, closed systems is inherently flawed, and that it is more appropriate to consider each lake as part of an open system. Recent RES surveys of some of the ‘active’ lake sites that do not show the classic lake-like reflectors support this view, suggesting that at those sites the lakes may periodically experience wholesale drainage or that they are very shallow. An alternative explanation is that the surface movement detected by altimetry is not a signal of lake drainage/filling but rather the surface expression of subglacial migration of packets of basal water or sediment flows facilitated by changes to subglacial hydrological routing (Wright & Siegert Reference Wright and Siegert2012, Siegert et al. Reference Siegert, Ross, Corr, Smith, Jordan, Bingham, Ferraccioli, Rippin and Le Brocq2014). This interpretation implies that in some areas the storage of water and sediment is relatively temporary, and driven by internal hydrological (re)organization; consequently, caution should be applied when interpreting surface uplift/subsidence as the location of a fixed ‘lake’ that drains and recharges with some periodicity. Either explanation for the mismatch appeals to considerable dynamism in the subglacial hydrological system.
The impact of subglacial lakes on vertical ice motion, in some cases through several km of ice, highlights their potentially pervasive influence on ice dynamics. Siegert & Bamber (Reference Siegert and Bamber2000), Pattyn et al. (Reference Pattyn, De Smedt and Souchez2004), Bell et al. (Reference Bell, Studinger, Shuman, Fahnestock and Joughin2007) and Peters et al. (Reference Peters, Blankenship, Carter, Kempf, Young and Holt2007) have all noted that subglacial lakes towards the ice sheet interiors coincide with ice stream onset regions and may, therefore, play a critical role in defining the configuration of drainage across Antarctica. This is particularly true in areas of East Antarctica where thick inland ice causes large areas of the bed to be at pressure melting point, allowing liquid water to collect in hydraulic potential lows caused by low ice surface slopes and rough subglacial topography. Of further significance, Stearns et al. (Reference Stearns, Smith and Hamilton2008) linked a 14-month acceleration of Byrd Glacier, East Antarctica, to the drainage of two subglacial lakes c. 200 km further upstream. To explain the acceleration (and subsequent deceleration) of this glacier, Stearns et al. (Reference Stearns, Smith and Hamilton2008) drew directly on established relationships between subglacial hydrological configurations and ice dynamics of temperate glaciers developed by Kamb (Reference Kamb1987). These papers have been especially important in underscoring that: i) subglacial water clearly has the capacity to travel long distances through drainage networks beneath the Antarctic ice sheet, and ii) it is the flow of subglacial water from one place to another, especially if there are sudden changes in volume, that may have the most profound influence on ice dynamics. The latter point is profoundly familiar to researchers with a background in temperate and Arctic polythermal glaciers and, more recently, the Greenland ice sheet, although in these cases the most variable and glaciodynamically significant water flows tend to be induced by surface melt penetrating to the bed, whereas in Antarctica alternative forcing mechanisms should be considered. Periodic subglacial lake drainage provides one such mechanism (Evatt et al. Reference Evatt, Fowler, Clark and Hulton2006, Pattyn Reference Pattyn2011).
Subglacial water away from lakes
This section will focus on observations and theories of subglacial water generation and flow beneath the Antarctic ice sheet, separate from the explicit study of subglacial lakes. A seminal programme of studies on the Siple Coast ice streams, initiated in the 1980s, provided some of the earliest observational groundwork for the existence of distributed water at the beds of ice streams and their tributaries. Using seismic techniques, Blankenship et al. (Reference Blankenship, Bentley, Rooney and Alley1986) presented the first evidence to show that the bed of an active ice stream, in this case Whillans Ice Stream, was composed of water-saturated sediments. Alley et al. (Reference Alley, Blankenship, Rooney and Bentley1989) demonstrated the crucial importance of this water in facilitating deformation of the underlying sediments and therefore fast flow in the overlying ice. Drilling to the bed of this ice stream, Engelhardt et al. (Reference Engelhardt, Humphrey, Kamb and Fahnestock1990) confirmed that the bed was at pressure melting point and that the subglacial water was highly pressurized. Subsequent experiments demonstrated that the subglacial water pressures (hence, inferred water flows) were highly variable (Engelhardt & Kamb Reference Engelhardt and Kamb1997). Part of the variability could be attributed to tidal influences at sites sufficiently proximal to the grounding line; but there was also considerable unexplained variability in water pressure, which, they argued, acted cumulatively to support continued fast flow of overlying ice. Parallel studies by Smith (Reference Smith1997a, Reference Smith1997b) and Vaughan et al. (Reference Vaughan, Smith, Nath and Le Meur2003) also derived seismic acoustic-impedance to argue for the existence of wet sediments beneath several additional West Antarctic ice streams, while King et al. (Reference King, Woodward and Smith2004) presented seismic evidence that water may be routed through small channels, or ‘canals’, along the Rutford Ice Stream bed. All of these studies, and further analysis of RES bed reflectivity, have shown that most ice streams, and many ice stream tributaries, are underlain by ice at pressure melting point, contrasting with surrounding regions commonly underlain by cold-based ice. Therefore, the presence of water is crucial to ice dynamics in many areas and for determining the current configuration of ice flow across Antarctica. However, the mechanisms by which the water is generated, and the specific processes by which it flows and impacts upon overlying ice dynamics, remain poorly understood. For example, the presence of water at the bed alone is not exclusively responsible for the fast flow of ice, as verified by the existence of water beneath the currently stagnated Kamb Ice Stream (Catania et al. Reference Catania, Conway, Gades, Raymond and Engelhardt2003). Moreover, the fast flow of the tributaries of Slessor Glacier, East Antarctica, appears to be initiated by ice deformation alone (Rippin et al. Reference Rippin, Bamber, Siegert, Vaughan and Corr2003), with subglacial water only becoming available farther downstream, presumably when frictional heat sources sufficiently elevate basal temperatures.
With supraglacial and englacial meltwater sources ruled out, sources of basal water beneath the Antarctic ice sheet must be derived predominantly from geothermal heating and pressure melting. Additional contributions may come from: i) frictional heating generated by ice motion over the subglacial substrate, ii) occasional release of water through the regelation process (Weertman Reference Weertman1957), and iii) (at a local level) drainage from subglacial lakes or reservoirs upstream. However, the distribution of geothermal heating beneath Antarctica is notoriously poorly constrained due to a dearth of observations. In the last decade, two modelled distributions of geothermal heat flux across the Antarctic bed have been derived: the first extrapolated from a global seismic model of the Earth’s crust and upper mantle (Shapiro & Ritzwoller Reference Shapiro and Ritzwoller2004) and the second derived from satellite magnetic measurements (Maule et al. Reference Maule, Purucker, Olsen and Mosegaard2005). Both have been used in ice sheet modelling to derive continent-wide maps of basal thermal conditions (Pattyn Reference Pattyn2010, Van Liefferinge & Pattyn Reference Van Liefferinge and Pattyn2013) but they differ in many details, rendering significant uncertainty to any interpretations of subglacial water distribution. At a broad level, both models show much greater geothermal heat flux across West Antarctica relative to East Antarctica, supporting (though not confirming) claims of active volcanism beneath the West Antarctic Ice Sheet (Blankenship et al. Reference Blankenship, Bell, Hodge, Brozena, Behrendt and Finn1993). Brief periods of volcanic activity may be responsible for producing surplus meltwater sufficient to induce excess basal slip in some localities (Vogel & Tulaczyk Reference Vogel and Tulaczyk2006, Corr & Vaughan Reference Corr and Vaughan2008), in effect aping the transient lake-drainage-triggered acceleration of Byrd Glacier reported by Stearns et al. (Reference Stearns, Smith and Hamilton2008). The importance of active volcanism to the overall pattern of ice dynamics in Antarctica remains debated.
To expand upon observational records of the distribution and processes of water flow beneath the Antarctic ice sheet, several teams from the 1980s onwards examined the properties of bed returns in RES data for signals of water and its variations, i.e. other than identifying just the obvious lake-like reflectors. Shabtaie et al. (Reference Shabtaie, Whillans and Bentley1987) argued that relative reflection amplitudes, after corrections for variations in ice thickness and taking into account possible variations in subglacial geology, varied inversely with freezing at the bed. Bentley et al. (Reference Bentley, Lord and Liu1998) later applied a revised version of this scheme to suggest high bed reflection amplitudes beneath Kamb Ice Stream evinced liquid water at its base, while low relative reflection amplitudes beneath neighbouring Engelhardt Ice Ridge were indicative of frozen till. Gades (Reference Gades1998) drew on these studies, and from radar work on temperate glaciers, to develop a range of ‘bed reflection powers’ for subglacial materials from frozen permafrost to liquid water, then applied this classification to the subglacial interface sounded across Siple Dome (Gades et al. Reference Gades, Raymond, Conway and Jacobel2000). This study showed that while the dome was frozen to the bed, the ice streams flanking it were underlain by water-saturated material. Subsequent RES surveying and recovery of bed reflection power (as quantified by the amplitudes of the bed-return waves) across a number of the Siple Coast ice streams, calibrated with observations of water content in nearby boreholes, confirmed the contention that the ice streams (including the currently stagnant Kamb Ice Stream) are underlain by wet sediment, while in the intervening ridges the water is frozen to the bed (Catania et al. Reference Catania, Conway, Gades, Raymond and Engelhardt2003, Peters et al. Reference Peters, Blankenship and Morse2005).
In recent years, the use of RES to search for water beneath the Antarctic ice sheet has been adopted as a standard part of any survey, and has been applied far beyond the Siple Coast region, into other parts of West Antarctica and across East Antarctica. Table I provides a summary of surveys and significant findings (see also example RES data presented in Fig. 4). In acquiring and analysing more recent data and, in some cases, retrospectively analysing older surveys, research has advanced from the simple binary exercise of distinguishing between ‘dry’ and ‘wet’ beds, and significant developments have been made. Firstly, now that modern RES systems are fully digitized, bed returns can be acquired at much higher resolution and the collected data analysed with much greater efficiency. Though data are always degraded by beam spreading, limiting our ability to acquire data at the resolution of many hypothesized subglacial system structures, the recovery of hydrological variability at finer resolution is much improved. Technical advances in data acquisition, such as synthetic aperture processing radio echo sounding platforms, have aided this development (Helière et al. Reference Helière, Lin, Corr and Vaughan2007, Peters et al. Reference Peters, Anandakrishnan, Alley and Smith2007). Synthetic aperture processing, used together with migration processing (inherited from seismic processing), reinstates energy to its true position in two- or three-dimensions, thereby increasing resolution. Secondly, the treatment of englacial attenuation, the loss of signal on its passage through the ice, has seen progress recently. Matsuoka (Reference Matsuoka2011) highlighted the risks of over-interpreting bed returns in the absence of correct treatment of englacial attenuation, especially where the ice-flow regime and subglacial topography are complex (see Matsuoka et al. Reference Matsuoka, MacGregor and Pattyn2010 for a review). Thirdly, information in the bed returns other than, or supplementary to, the amplitude of the bed return is being used. For example, Murray et al. (Reference Murray, Corr, Forieri and Smith2008) used coincident radar and seismic profiles to recover subglacial conditions, while Schroeder et al. (Reference Schroeder, Blankenship and Young2013) used specular anisotropy of bed returns to image sub-resolution hydrological features largely independently of the effects of englacial attenuation.
Table I Summary of radioglaciological studies in which bed-reflection power (bed reflectivity) has been acquired across parts of the Antarctic ice sheet organized chronologically by survey date. Traverses between locations are denoted by hyphenation. Only Antarctic-focused studies from the last 15 years specifically concerned with the basal reflectivity of grounded ice are listed. Bright reflections have been used to (semi-)qualitatively delineate subglacial lakes since the 1960s and such studies are not included here.

BRP=bed-returned power, DMLWasa=Dronning Maud Land Wasa expedition, EAIS=East Antarctic Ice Sheet, EPICA=European Project for Ice Coring in Antarctica, KIS=Kamb Ice Stream, MSGL=mega-scale glacial lineations.
Several researchers have sought to model flow routes beneath the ice sheet to develop the theory that water flows for great distances beneath the Antarctic ice sheet. Rémy & Legrésy (Reference Rémy and Legrésy2005) performed hydrological network analysis on high-resolution ice surface data over Wilkes Land, East Antarctica, mapping an inferred subglacial network that linked several known subglacial lakes. A significant assumption in their analysis was that surface topography on a broad-scale reflects the subglacial topography; though later work demonstrated that this only holds true under certain conditions (De Rydt et al. Reference De Rydt, Gudmundsson, Corr and Christoffersen2013). Siegert et al. (Reference Siegert, Le Brocq and Payne2007) modelled subglacial water routing across Antarctica using hydropotential methodology (Shreve Reference Shreve1972), which requires knowledge of the ice surface and the bed. Le Brocq et al. (Reference Le Brocq, Payne, Siegert and Alley2009) used a similar method when developing a subglacial water flow component for ice sheet modelling. The results of these exercises must be viewed with a confidence relative to our knowledge of bed topography and conditions across different regions, and in the context that small changes to the ice surface, such as external forcings or internal instabilities, can exert significant influences on subglacial water flowpaths (Wright et al. Reference Wright, Siegert, Le Brocq and Gore2008). Carter et al. (Reference Carter, Blankenship, Young, Peters, Holt and Siegert2009b) modelled the probable flow mechanism and impact on ice mass budget of the Adventure Lakes drainage event (described by Wingham et al. Reference Wingham, Siegert, Shepherd and Muir2006). The authors suggested that the water flow was probably distributed along a broad, shallow water system, perhaps through basal sediments, and that this flowpath delayed the arrival of water to downstream points by approximately 12 months. Carter & Fricker (Reference Carter and Fricker2012) modelled the subglacial water flows to the Siple Coast. The aforementioned studies have all assumed, or inferred, that most subglacial water flows through distributed drainage systems beneath the ice sheet. However, Le Brocq et al. (Reference Le Brocq, Ross, Griggs, Bingham, Corr, Ferraccioli, Jenkins, Jordan, Payne, Rippin and Siegert2013) observed supraglacial channels close to the grounding line on the surfaces of a number of ice shelves, and inferred that they may originate from concentrated outflows of subglacial water flowing along channelized flowpaths beneath the inland ice. Should this be true, and further data are required as confirmation, it challenges the way in which subglacial flows have been modelled. In support of this, Schroeder et al. (Reference Schroeder, Blankenship and Young2013) demonstrated that, beneath Thwaites Glacier, the specularity of radar returns changed with respect to whether flightlines were parallel or perpendicular to ice flow, indicating the presence of distributed ‘canals’ and upward incising channels in the upstream and downstream parts of the bed, respectively.
Extensive surveys over parts of East Antarctica are proffering further insight into subglacial water routing. For example, Wright et al. (Reference Wright, Young, Roberts, Schroeder, Bamber, Dowdeswell, Young, Le Brocq, Warner, Payne, Blankenship, van Ommen and Siegert2012) presented a suite of evidence from an aerogeophysical survey over the 287 000 km3 Aurora Basin to demonstrate that subglacial lakes near the Dome C ice divide probably discharge through Aurora Basin to the coast via Totten Glacier. A survey of the Gamburtsev Subglacial Mountains in East Antarctica has also provided a glimpse of the subglacial hydrological network in the deep interior (Wolovick et al. Reference Wolovick, Bell, Creyts and Frearson2013). Water is predominantly routed along a system of alpine overdeepenings created during the early growth phase of the East Antarctic Ice Sheet, and the drainage networks follow valley floors either uphill or downhill depending on the gradient of the ice sheet surface. Where the water is forced uphill along the valley floor by the ice surface gradient, it terminates in or near distinct and sometimes very large (up to 25% of the ice column) plumes of refrozen basal ice which have strong potential to alter basal ice rheology and fabric, and are implicated in thickening the overlying ice column (Bell et al. Reference Bell, Ferraccioli, Creyts, Braaten, Corr, Das, Damaske, Frearson, Jordan, Rose, Studinger and Wolovick2011). These observations suggest that supercooling of basal water remains a significant, and perhaps underestimated, component of the subglacial water budget across Antarctica, by acting to recover water that would otherwise eventually drain to the margins.
Despite improved knowledge of the Antarctic subglacial hydrology, there remain few observations targeted towards monitoring changes in the subglacial hydrological system. The ‘active’ lake studies drew attention to the instability of subglacial water sources and flows (e.g. Gray et al. Reference Gray, Joughin, Tulaczyk, Spikes, Bindschadler and Jezek2005, Wingham et al. Reference Wingham, Siegert, Shepherd and Muir2006, Fricker et al. Reference Fricker, Scambos, Bindschadler and Padman2007), but the majority of evidence is provided indirectly by glaciodynamic instabilities that are probably associated with unstable water flows in the subglacial drainage system. A clear example of a glaciodynamic instability probably related to changes in subglacial water flow is provided by Kamb Ice Stream. Formerly fast-flowing like the other Siple Coast ice streams, it switched to a slow flow state more than a century ago (Retzlaff & Bentley Reference Retzlaff and Bentley1993). Anandakrishnan & Alley (Reference Anandakrishnan and Alley1997) surmised that the stagnation of flow resulted from piracy of much of the ‘lubricating’ subglacial water to neighbouring Whillans Ice Stream, allowing localized ‘sticky spots’ to gain eminence sufficient to deactivate fast flow. Catania et al. (Reference Catania, Scambos, Conway and Raymond2006) presented further evidence that around the same time the margin of Kamb Ice Stream shifted inwards, resulting in a narrowing of the ice stream trunk and discharge capacity by c. 30%. Other authors have shown that with lower water fluxes much of the remaining water has been susceptible to basal freeze-on (Christoffersen & Tulaczyk Reference Christoffersen and Tulaczyk2003, Vogel et al. Reference Vogel, Tulaczyk, Kamb, Englehardt, Carsey, Behar, Lane and Joughin2005). Examination of sediments recovered from beneath Kamb Ice Stream (Engelhardt & Kamb Reference Engelhardt and Kamb2013), ancillary evidence from ice surface flow-lines extending across the ice shelf (Hulbe & Fahnestock Reference Hulbe and Fahnestock2007) and modelling (Christoffersen et al. Reference Christoffersen, Tulaczyk and Behar2010, Catania et al. Reference Catania, Hulbe, Conway, Scambos and Raymond2012, Van der Wel et al. Reference Van der Wel, Christofferson and Bougamont2013) suggest that over long timescales Kamb Ice Stream may undergo cycles of activity and stagnation; the cause of the initial piracy or recovery of water flows beneath the ice stream remains enigmatic. Evidence of ice stream stagnation probably due to water piracy has also been reported from Carlson Inlet/Rutford Ice Stream (Vaughan et al. Reference Vaughan, Corr, Smith, Pritchard and Shepherd2008) and in the Ragnhild Glacier system of East Antarctica (Pattyn et al. Reference Pattyn, De Brabander and Huyghe2005).
Seismic techniques have been successfully deployed to observe changes in the subglacial hydrological system beneath Antarctica. For example, repeat active-seismic surveys on Rutford Ice Stream have shown switches in hydrological behaviour at the same site surveyed at six/seven year intervals (Smith et al. Reference Smith, Murray, Nicholls, Makinson, Adalgeirsdóttir, Behar and Vaughan2007). In another study, Winberry et al. (Reference Winberry, Anandakrishnan and Alley2009) used passive seismic sensors to record a crack opening towards the bed and to ‘hear’ rushing water for a period of ten minutes at the bed of MacAyeal Ice Stream. These studies offer a tantalizing hint of the potential rapidity with which changes take place in the subglacial hydrological system beneath Antarctica, but improved knowledge requires more extensive deployment in terms of both spatial spread and repeat measurements.
Grand challenges
In the last decade, due to technological advances in remote sensing and exploratory geophysics, coupled with improved theoretical conceptions of subglacial processes beneath the major ice sheets, vast improvements have been made in our knowledge of the ways in which subglacial water is generated, stored, flows beneath and ultimately leaves the Antarctic ice sheet. Some of the grand challenges that now lie ahead are identified and discussed below.
Technological improvements and the expansion of the observational base
To date, technology has been key to observing subglacial hydrological phenomena, whether using geophysical techniques to image the basal environment (e.g. Blankenship et al. Reference Blankenship, Bentley, Rooney and Alley1986, Woodward et al. Reference Woodward, Smith, Ross, Thoma, Corr, King, King, Grosfeld, Tranter and Siegert2010), improving drilling to access it (e.g. Siegert et al. Reference Siegert, Kennicutt II and Bindschadler2011), or in developing new ways of interpreting subglacial hydrological behaviour from spaceborne remote sensing observations of the ice surface (e.g. Fricker et al. Reference Fricker, Scambos, Bindschadler and Padman2007). Regarding the latter, continued observations of ice surface change, such as by CryoSat (McMillan et al. Reference McMillan, Corr, Shepherd, Ridout, Laxon and Cullen2013) and IceSAT/IceBridge programs (Fricker et al. Reference Fricker, Scambos, Carter, Davis, Haran and Joughin2010), offer efficient data capture, could be targeted over predicted subglacial lakes and water flowpaths (cf. Livingstone et al. Reference Livingstone, Clark, Woodward and Kingslake2013), and calibrated with field measurements over inferred lakes (e.g. Christianson et al. Reference Christianson, Jacobel, Horgan, Anandakrishnan and Alley2012, Siegert et al. Reference Siegert, Ross, Corr, Smith, Jordan, Bingham, Ferraccioli, Rippin and Le Brocq2014). At the same time, it is important not to neglect observational programmes on and over the ice that could pick up subglacial hydrological changes too subtle to be detected by spaceborne remote sensing, but which may still significantly impact on ice dynamics. Radar instrumentation and processing is one area in which advances have been particularly apparent over the last decade, driven in part by the NASA IceBridge program. Research into beam-forming processing techniques offers the exciting potential for a multi-antennae array to survey swaths of the ice bed, similar to bathymetric multi-beam sonar surveying, vastly improving the efficiency of radar surveys (Paden et al. Reference Paden, Akins, Dunson, Allen and Gogineni2010, Wu et al. Reference Wu, Jezek, Rodriguez, Gogineni, Rodriguez-Morales and Freeman2011). The development and application of ultra-wide-band sensors (e.g. Laird et al. Reference Laird, Blake, Matsuoka, Conway, Allen, Leucschen and Gogineni2010) offer similar prospects for extracting basal properties at an increasing resolution. These advances will require an increased understanding of how radar signals interact with the ice bed interface, in particular with shallow lake environments (e.g. Gorman & Siegert Reference Gorman and Siegert1999). One method to decrease this ambiguity is through the increased use of reflectivity modelling with both real and synthetic datasets (e.g. MacGregor et al. Reference MacGregor, Anandakrishnan, Catania and Winebrenner2011). A significant challenge for radar surveying lies in improving the treatment of englacial attenuation (e.g. Matsuoka et al. Reference Matsuoka, MacGregor and Pattyn2010, Matsuoka Reference Matsuoka2011, Ashmore et al. Reference Ashmore, Bingham, Hindmarsh, Corr and Joughin2014) and improving our ability to discriminate between water at the bed versus contrasts in subglacial geology. Undertaking radar surveys in tandem with seismic surveys (e.g. Murray et al. Reference Murray, Corr, Forieri and Smith2008) or potential-field surveying (e.g. Smith et al. Reference Smith, Jordan, Ferraccioli and Bingham2013) offers promise for these objectives. Completing combined seismic and radar surveys over larger areas would be aided significantly by new seismic instrumentation. Truck-mounted swept-frequency sources (Vibroseis), as are used in terrestrial hydrocarbon exploration, with snow streamer receivers (Hofstede et al. Reference Hofstede, Eisen, Diez, Jansen, Kristoffersen, Lambrecht and Mayer2013), offer a more efficient method for seismic data collection in the future. While a programme of direct-access measurements of hydrology at the ice base would offer the most direct observations, it would be expensive and logistically challenging. Therefore, in the medium-term, our best hope of acquiring observations to improve understanding of subglacial hydrology remains with continuing geophysical survey programmes, especially the use of radar to identify hydrological variability.
Improved understanding of the geothermal heat flux in Antarctica
With supraglacial meltwater inputs to the subglacial system precluded in Antarctica, one of the root causes of subglacial hydrological variability is probably spatial and temporal variations in the generation of subglacial meltwater at the ice sheet base. Therefore, a key objective must be to obtain an improved understanding of a chief control on subglacial meltwater generation, namely the geothermal heat flux, thereby predicting the magnitude of subglacial melt from different regions beneath the ice sheet. Spatial maps of geothermal heat flux based on global seismic modelling (Shapiro & Ritzwoller Reference Shapiro and Ritzwoller2004) or satellite magnetic measurements (Maule et al. Reference Maule, Purucker, Olsen and Mosegaard2005) are currently being used, in part, to derive first-order estimates of subglacial melt (e.g. Pattyn Reference Pattyn2010). However, without direct measurements to calibrate these maps, and considering that in some locations they differ enormously in their distributions, there remains considerable uncertainty over the magnitude of geothermal heat flux in many locations. Ultimately, an integrated multi-dataset-based geothermal heat flux model is required for Antarctica, similar to that produced for Greenland (cf. Rogozhina et al. Reference Rogozhina, Hagedoom, Martinec, Fleming, Soucek, Greve and Thomas2012). Additional datasets that could be used to support such an objective include airborne- and satellite-acquired gravity data (e.g. Vaughan et al. Reference Vaughan, Smith, Nath and Le Meur2003), as well as calibration from rapid-access drilling to the bed of the ice sheet in as many locations as possible, and the use of subglacial lakes and other radar-imaged ‘wet’ basal returns to calibrate locations of warm-based ice.
Improved understanding of subglacial water flowpaths and mechanisms
While most modelling of subglacial water flowpaths in Antarctica has assumed that subglacial water follows distributed flowpaths, the findings of Le Brocq et al. (Reference Le Brocq, Ross, Griggs, Bingham, Corr, Ferraccioli, Jenkins, Jordan, Payne, Rippin and Siegert2013) suggest that channelized flowpaths can exist from some way inland of the grounding line. Hence, a challenge ahead is to discern what conditions are required to keep large subglacial channels open beneath ice streams, and understand the impact this may have on overlying ice dynamics. As part of engaging with this challenge, a requirement will be to understand how the subglacial drainage system may evolve beneath an ice stream in the absence of supraglacial forcing, which is known to be the chief forcing mechanism behind subglacial drainage system evolution in alpine and Arctic systems (cf. Nienow et al. Reference Nienow, Sharp and Willis1998, Bartholomew et al. Reference Bartholomew, Nienow, Mair, Hubbard, King and Sole2010). Subglacial lakes, in particular the regular or irregular drainage from upstream to downstream locations and ultimately to the ice margin, represent one mechanism for initiating the large subglacial water flows one might conceive are necessary for channelization, but how channels remain open underneath deep ice for periods exceeding the length of each drainage event is unknown. Repeat seismic surveys of Rutford Ice Stream have been informative on the changing subglacial environment (Smith et al. Reference Smith, Murray, Nicholls, Makinson, Adalgeirsdóttir, Behar and Vaughan2007) but remain time-consuming and below the temporal resolution of hydrological changes. Geophysical installations, as have been performed in Alpine environments (Kulessa et al. Reference Kulessa, Booth, Hobbs and Hubbard2008), may provide information on subglacial hydrological dynamics at higher temporal resolution. Passive seismic and GPS installations, in particular, may be useful for elucidating the temporal aspect of subglacial drainage and the interaction of water with the substrate (e.g. Winberry et al. Reference Winberry, Anandakrishnan and Alley2009, Reference Winberry, Anandakrishnan, Wiens, Alley and Christianson2011).
Determining the role of subglacial water in ice sheet mass balance
The demonstrable dynamism in subglacial water beneath Antarctica throws into doubt the previously unstated assumption that the role of subglacial water in ice sheet mass balance is negligible. In interior regions, for example, local mass balance may be affected by the widespread phenomenon of substantial volumes of subglacial melt freezing back on to the underside of the ice at bedrock perturbations to form large blocks of accreted ice. This not only removes water that would otherwise have drained downstream, but also affects the internal flow properties of the ice (Bell et al. Reference Bell, Ferraccioli, Creyts, Braaten, Corr, Das, Damaske, Frearson, Jordan, Rose, Studinger and Wolovick2011). On the scale of an ice sheet, the amount of water that is lost to groundwater flow beneath the ice is unknown, and there is limited knowledge of typical volumes of water lost to outburst events at the margin. There is only one published observation of an active subglacial outburst from the Antarctic ice sheet (Law Dome, East Antarctica; Goodwin Reference Goodwin1988), and it is probable that most meltwater leaves the ice sheet unobserved across the grounding line and directly into the ocean. One aspect of subglacial water routing, not considered until now, was described by Uemera et al. (Reference Uemura, Taniguchi and Shibuya2011) who measured an upward freshwater groundwater flux offshore of East Antarctica, which may have been re-emerging subglacial meltwater. New and innovative ways are required to identify probable water outflows from the Antarctic ice sheet and their impact on the ice sheet mass balance.
Uniting geomorphology and process-glaciology to consolidate understanding
For many years, the labyrinthine landscape of subglacially-carved channels exposed in the Dry Valleys of East Antarctica, studied by David Sugden and colleagues (cf. Sugden & John Reference Sugden and John1976 p. 1, Sugden et al. Reference Sugden, Bentley and Ó Cofaigh2006), has presented clear evidence not only that subglacial water flows occur beneath the Antarctic ice sheet, but that they occur with sufficient magnitudes to scour their signal clearly across the subglacial surface, even where that surface consists of hard rock. Offshore from the present-day Antarctic coastline, across the continental shelf which lay under an expanded ice sheet during the Last Glacial Maximum, there is ample evidence of abundant channels and cavities carved into the subglacial surface by inferred subglacial drainage pathways (e.g. Nitsche et al. Reference Nitsche, Gohl, Larter, Hillenbrand, Kuhn, Smith, Jacobs, Anderson and Jakobsson2013). The existence of these signals across the landscape invites the question: how effective is subglacial drainage beneath the Antarctic ice sheet as an active erosional agent, and how effective is it at transporting material from the ice sheet interior to the margin? More generally, it serves as the latest refresher that there is much to be gained from continual knowledge transfer and exchange of ideas between the glacial geomorphology and process-glaciology communities.
Acknowledgements
DWA and RGB wish to thank the University of Aberdeen College of Physical Sciences, the Scottish Alliance for Geoscience, Environment and Society, and the Royal Astronomical Society for financial support towards the production of this paper. We also thank David Rippin, an anonymous reviewer, and the scientific editor Andrew Mackintosh for thorough reviews that benefitted the final manuscript.