1. Introduction
Most of the early discoveries of Ediacaran fossils were serendipitous (Richter, Reference Richter1955; Ford. Reference Ford1958; Anderson & Misra, Reference Anderson and Misra1968; Keller & Fedonkin, Reference Keller and Fedonkin1976; Minicucci, Reference Minicucci2017), but Reg Sprigg (1919–1994) was actually looking for early animals when he found the holotype of Ediacaria flindersi (Fig. 1) at Ediacara in March 1946 (Sprigg, Reference Sprigg1947, Reference Sprigg1988). Furthermore, he found just what he and the World had expected: a jellyfish from the ‘age of jellyfishes’ (Sprigg, Reference Sprigg1949, p. 97) – a concept that may have been influenced by C. D. Walcott’s monograph Fossil Medusae (Walcott, Reference Walcott1898), the report of a probable jellyfish, Brooksella canyonensis, from the Neoproterozoic Nankoweap Formation of the Grand Canyon (Bassler, Reference Bassler1941; Glaessner, Reference Glaessner1962) and possibly by Gürich’s (Reference Gürich1933) report of half a jellyfish (Paramedusium africanum) from the Nama beds of southern Africa. Sprigg was undoubtedly also encouraged by Kenneth Caster’s comprehensive report of a new fossil jellyfish (Kirklandia texana) from the Cretaceous of Texas (Caster, Reference Caster1945), which he referenced in 1949. Almost all of these objects are now thought to be either trace or pseudofossils (Fürsich & Kennedy, Reference Fürsich and Kennedy1975; Runnegar & Fedonkin, Reference Runnegar, Fedonkin, Schopf and Klein1992). Nevertheless, Sprigg’s jellyfish hypothesis for the nature and mode of preservation of some of the most abundant Ediacaran fossils was widely influential for nearly 50 years, both in Australia (Glaessner, Reference Glaessner1961, Reference Glaessner1984; Wade, Reference Wade1972 b; Oliver & Coates, Reference Oliver, Coates, Boardman, Cheetham and Rowell1987; Jenkins, Reference Jenkins1989) and in the Soviet Union (Fedonkin, Reference Fedonkin1985 a, Reference Fedonkin1987). How did Sprigg’s jellyfish hypothesis arise and remain viable for so long? What other major hypotheses have been advanced to explain the Ediacara fauna? How have tradition, national proclivities and innovative thinking helped or hindered our understanding of the true nature of the Ediacara biota? These are some of the matters that are explored in this article.

Fig. 1. Martin Glaessner’s illustration of Sprigg’s jellyfish hypothesis for the nature of the common Ediacaran fossils and their environment of deposition at Ediacara, South Australia plus his own Pennatulacea hypothesis, including a manifestation of Ford’s chimera (Fig. 2, bottom right). Insert at upper left is Sprigg’s reconstruction of the scyphozoan jellyfish Ediacaria flindersi. After Glaessner (Reference Glaessner1961) and Sprigg (Reference Sprigg1949, Fig. 3B); © Bunji Tagawa 1961, republished with permission and with a Creative Commons Licence, respectively.
2. Ediacaran hypotheses
2.a. Sprigg’s jellyfish hypothesis (1947+)
Sprigg’s exposure to dehydrating jellyfish on Adelaide’s fine beaches (Sprigg, Reference Sprigg1989) preconditioned his assignment of Ediacaria and other discoidal ‘medusoids’ to the cnidarian classes Hydrozoa and Scyphozoa. Not realizing that the invariably convex discs were on bed bases rather than bed tops, he saw them as jellyfish stranded by tides. This powerful imagery was adopted by Martin Glaessner when he moved to the University of Adelaide and began working on the Ediacara fauna (Fig. 1) and was inherited by Glaessner’s successor – Richard Jenkins – and Glaessner’s students, despite clear evidence for bed base preservation and subtidal deposition (Gehling, Reference Gehling2000; McMahon et al. Reference McMahon, Liu, Tindal and Kleinhans2021). It was Jim Gehling’s reinterpretation of the environment of deposition of the Ediacara Member (Gehling, Reference Gehling2000) and his work with Guy Narbonne on the true nature of Aspidella Billings, Reference Billings1872 (Gehling et al. Reference Gehling2000) that ultimately killed the jellyfish hypothesis. Nevertheless, before it died Ediacaran ‘medusoids’ had been confidently placed in most extant and many extinct medusozoan classes: Hydrozoa, Scyphozoa, Cubozoa, Conulata, Dipleurozoa, Cyclozoa, Inordozoa, Trilobozoa (Sprigg, Reference Sprigg1949; Harrington & Moore, Reference Harrington and Moore1955; Glaessner & Wade, Reference Wade1971; Wade, Reference Wade1972 b; Jenkins, Reference Jenkins1984; Sun, Reference Sun1986; Fedonkin, Reference Fedonkin1987; Oliver & Coates, Reference Oliver, Coates, Boardman, Cheetham and Rowell1987). It is sobering to appreciate that four classic Ediacaran jellyfish – Ediacaria Sprigg, Reference Sprigg1947 (Scyphozoa), Cyclomedusa Sprigg, Reference Sprigg1947 (Cyclozoa), Protodipleurosoma Sprigg, Reference Sprigg1949 (Hydrozoa) and Irridinitus Fedonkin, Reference Fedonkin, Velikanov, Aseeva and Fedonkin1983 (Inordozoa) – are no more than preservational variants of the holdfast of a single species of Arborea Glaessner & Wade, Reference Glaessner and Wade1966 (Ivantsov, Reference Ivantsov2016).
2.b. Glaessner’s Pennatulacea hypothesis (1959+)
Martin Glaessner (1906–1989) and Curt Teichert (1905–1996) were the first palaeontologists to view and collect Ediacaran fossils in South Australia. Both examined the holotype of Ediacaria flindersi during an ANZAAS meeting in Adelaide in August 1946, and Teichert travelled with Sprigg and his wife to Ediacara in 1947. However, it was Glaessner rather than Teichert who seized the opportunity after he moved to the University of Adelaide in 1950.
Sprigg’s jellyfish hypothesis was supplemented by two major embellishments during the 1950s. Remarkably they came from discoveries in all three of the now recognized Ediacaran associations: Avalon (Charnwood), White Sea (Ediacara) and Nama (Namibia) (Gehling & Droser, Reference Gehling and Droser2013). Working with newly collected material from Namibia, Rudolf Richter (1881–1957) showed that Pteridinium simplex Gürich, Reference Gürich1933, which had previously been thought to resemble a fern, was constructed of three separate vanes, each being formed of tubular modules (Richter, Reference Richter1955). As the only living animals having this kind of triradial geometry are gorgoniid octocorals, the cnidarian or ‘coelenterate’ affinity of Ediacarans received additional support. Then in 1958, Trevor Ford (1925–2017) reported Roger Mason’s discovery of Charnia and Charniodiscus in England (Fig. 2). At the time, Ford preferred an algal rather than an animal affinity for these two fronds but reluctantly yielded to a letter Glaessner published soon afterwards in Nature entitled ‘Precambrian Coelenterata from Australia, Africa and England’ (Glaessner, Reference Glaessner1959 b; Ford, Reference Ford1980). According to Glaessner, the taxa we now know as rangeomorphs and arboreomorphs were pennatulacean octocorals and thus both highly derived cnidarians but also colonies that had achieved a high degree of integration that allowed them to act as collective ‘individuals’ (Dewel et al. Reference Dewel, Dewel and McKinney2001). This concept of complex coloniality was elaborately developed by Hans D. Pflug as the Petalonamae hypothesis for the common taxa of the Nama Association (Pflug, Reference Pflug1966, Reference Pflug1970, Reference Pflug1971, Reference Pflug1972 a).

Fig. 2. Trevor Ford’s chimera based on the frond of the rangeomorph Charnia and the holdfast of the arboreomorph Charniodiscus bridges the gulf between the two clades; both from the North Quarry of the Charnwood Golf Course, Leicestershire, UK, a locality discovered by Roger Mason in 1957 (Ford, Reference Ford1958, Fig. 3); © Yorkshire Geological Society 1958, republished with permission.
2.c. Pflug’s Petalonamae hypothesis (1966+)
Glaessner had a couple of opportunities to doubt his own pennatulacean hypothesis but his belief in it may have overwhelmed his objectivity: a lesson for us all. As a native German speaker, he had read Gürich and Richter’s descriptions of Pteridinium carefully and was intimately familiar with fine specimens from Aar figured by Richter (Reference Richter1955), which he compared with small collections from the same site lent to him by the Geological Survey of South Africa and the Museum of Southwest Africa, Windhoek. Even so, he made no mention of the possibility of a third vane and instead emphasized similarities between Pteridinium and ‘Rangea’ (= Arborea), including his erroneous idea that both had primary and secondary orders of ribbing. He concluded that Pteridinium was a member of the Pennatulacea belonging to Richter’s extinct family Pteridiniidae, incertae sedis (Glaessner, Reference Glaessner1959 a, Reference Glaessner1963).
The second opportunity followed Mary Wade’s 1964 discovery of a few fragments of Pteridinium simplex in a mass flow channel deposit at Ediacara. This Nama-style preservation was unique for South Australia, and neither Glaessner nor Wade seems to have realized that their best specimen (Glaessner & Wade, Reference Glaessner and Wade1966, pl. 101, figs 1–3) shows clear evidence for three vanes. Unsurprisingly, they also concluded that Pteridinium and Rangea, as well as Arborea, were members of the Pennatulacea. However, only a few months earlier, Pflug (Reference Pflug1966) had described another small collection from Aar Farm and had begun to build a radically different hypothesis based initially on shared features of Pteridinium and his new genus, Ernietta (Fig. 3).

Fig. 3. (a) Ernietta plateauensis, Lower Member, Wood Canyon Formation, near Johnnie, Nevada, USA, specimen collected by Robert Horodyski, UCLA L7333-4; scale bar = 1 cm. (b, c) 3D models of Ernietta with tapered growing module tips and plane of symmetry parallel to the axial seam. (d) Model of the base of Ernietta shown in (c) has been reduced to nearly zero thickness in the Z direction to illustrate the topological relationship between (c) Ernietta and (d) Phyllozoon.
For the Petalonamae hypothesis, Pflug merged the morphology of average specimens of Ernietta with deformed U-shaped individuals of Pteridinium that had been transported and buried by storm surge sands at the Aar Lagerstätte locality (Meyer et al. Reference Meyer, Elliott, Wood, Polys, Colbert, Maisano, Vickers-Rich, Hall, Hoffman, Schneider and Xiao2014 b, figs 3–5; Pflug, Reference Pflug1970, pl. 23, figs 1, 3; Richter, Reference Richter1955, pl. 7, Fig. 11). In his mind – and if I have it right – the bag-shaped body (corpus) was formed of leaves or vanes (petaloids) made from co-aligned tubular modules, each of which housed an individual (persona) of the colony. Branching of the modules was sympodial rather than dichotomous, opposite, alternate or monopodial, resulting in zig-zag junctions between adjacent petaloids. In some forms the petaloids were replicated like the layers of an onion and adjacent petaloids or sets of petaloids curved to enclose a ‘petaloid cavity’ (centrarium). Channels between sets of petaloids (petalodia) served as gullets. Clade disparity was summarized using five shape categories and five alternative positions for the ‘ingestion aperture’ (Pflug, Reference Pflug1972 a, table 1).
Richard Jenkins demolished this house of cards with the pithy statement: ‘One of us (Jenkins) has examined Pflug’s material and considers that all the specimens he refers to as the “Erniettomorpha” belong to a single genus and species, Ernietta plateauensis Pflug’ (Jenkins et al. Reference Jenkins, Plummer and Moriarty1981, p. 71). Pflug’s theories would retain only academic interest except for the fact that he was a fine observer, so his drawings and descriptions are a major resource. However, maybe his concept of a centrarium is not as crazy as it seems and is worth a second look?
Gibson et al. (Reference Gibson, Rahman, Maloney, Racicot, Mocke, Laflamme and Darroch2019, Reference Gibson, Furbish, Rahman, Schmeeckle, Laflamme and Darroch2021) built a life-sized numerical model of an Ernietta from Aar and then used computational fluid dynamics and flume experiments with a 3D-printed version of the flask-like model to investigate how Ernietta might have used ambient water motion in feeding. They concluded that the observed gregarious growth allowed nutrient-rich water to preferentially enter the body cavity (Pflug’s centrarium), which was ‘likely the location of nutrient acquisition’ (Gibson et al. Reference Gibson, Furbish, Rahman, Schmeeckle, Laflamme and Darroch2021, p. 146). This process of an animal co-opting a piece of the external environment to develop a metabolically useful internal body cavity may be widespread. It was or is realised to lesser or greater extents in the atria of sponges and archaeocyaths, the cnidarian coelenteron, the bilaterian gut, the molluscan mantle cavity, the water vascular system of echinoderms, the chordate branchial basket, the marsupial pouch and the human lung. We could even extend the concept to the covid pod, if we were to go beyond the body. Thus, the centrarium of Ernietta could be part of a pathway to the coelenteron, as discussed in Section 6.c.
Pflug thought that the ancestral petalonamid might have resembled the colonial ciliate Zoothamnium (e.g. Bright et al. Reference Bright, Espada-Hinojosa, Volland, Drexel, Kesting, Kolar, Morchner, Nussbaumer, Ott, Scharhauser, Schuster, Zambalos and Nemeschkal2019). From there he saw a transition through Arborea and Charnia to Rangea, Pteridinium and Ernietta with much complexity added by preservational variants of each type. Here, I limit Petalonamae to three clades, Arboreomorpha, Rangeomorpha and Erniettomorpha (Erwin et al. Reference Erwin, Laflamme, Tweedt, Sperling, Pisani and Peterson2011), and treat dickinsoniomorphs under ‘Proarticulata’ (contra Hoyal Cuthill & Han, Reference Hoyal Cuthill and Han2018).
2.d. Seilacher’s Vendozoa hypothesis (1983+)
Dolf Seilacher (1925–2014) was struggling with understanding the Ediacaran organisms as early as 1976, when we began the discussion during a trip to the trace fossil-rich marine Permian of the southern Sydney Basin, initiated and organized by Bruce McCarthy, during the International Geological Congress (Cooper & Branagan, Reference Cooper and Branagan2015). At that time, Seilacher thought that they may have been megascopic prokaryotes. I remember well his subsequent presentation at the annual meeting of the Geological Society of America in Indianapolis in 1983, where he aired the Vendozoa concept, and Stephen Jay Gould’s enthusiastic reaction to it, despite the fact that Gould (1941–2002) was suffering severely from treatment for mesothelioma. The rest is history, but Gould served well as Seilacher’s bulldog in promoting the vendozoan hypothesis (Gould, Reference Gould1984).
Seilacher tried to place all of the soft-bodied Ediacaran organisms in a single clade, but even he had to allow some exceptions, most notably for the ‘sand corals’ (Psammocorallia) and the trace fossils (Seilacher, Reference Seilacher1989, Reference Seilacher1992). Originally conceived as something akin to stem lineage animals, vendozoans soon became vendobionts with their affinities transferred to the rhizopodan protists, specifically foraminiferans and xenophyophores (Seilacher, Reference Seilacher1992, Reference Seilacher2003). These kinds of alveolates are now far removed from the Opisthokonta, which includes the animals and fungi, but their supergroup (TSAR) is closer to the Archaeplastida (a.k.a. ‘plants’) than to the supergroup containing the opisthokonts (Amorphea; Burki et al. Reference Burki, Roger, Brown and Simpson2020). In that sense, both Petalonamae and Vendobionta conform to the notion of an extinct kingdom somewhere between animals and plants, which was what Pflug thought.
Other aspects of the vendozoan hypothesis can also be traced to Pflug’s ideas, although the Petalonamae were specifically excluded from the original proposal (Seilacher, Reference Seilacher1989, p. 237). Both Pflug and Seilacher emphasized the modular construction for tubular units as the unifying feature of this extinct clade and the mechanism that allowed growth to proceed to larger body sizes than had previously been possible.
2.e. Fedonkin’s Proarticulata hypothesis (1985+)
The holotype of Dickinsonia is incomplete so, with the jellyfish hypothesis in mind, Sprigg reconstructed it as being ‘symmetrical across both longitudinal and transverse planes’ (Sprigg, Reference Sprigg1947, p. 222). Even though this was clearly untrue after Sprigg’s second description of the fauna (Sprigg, Reference Sprigg1949), Harrington & Moore (Reference Harrington and Moore1955) still made Dickinsonia the only known example of their new coelenterate class Dipleurozoa. Perceptively, Glaessner thought that Dickinsonia ‘resembles certain worms more than any coelenterate’ (Glaessner, Reference Glaessner1958, Reference Glaessner1959 a; Glaessner & Daily, Reference Glaessner and Daily1959, p. 379). This idea was firmed up soon afterwards using comparisons of Dickinsonia and Spriggina Glaessner, Reference Glaessner1958 with the highly derived extant polychaetes Spinther and Tomopteris, respectively (Glaessner, Reference Glaessner1959 a, Reference Glaessner1961). Perhaps stimulated by Sidnie Manton’s (1902–1979) lead article on Spinther in the new Journal of Natural History (Manton, Reference Manton1967), Mary Wade (1928–2005) promoted Dickinsonia as a primitive polychaete ‘derived from ancestors with biramous parapodia and a more normal, elongate shape’. This idea was falsified by Runnegar (Reference Runnegar1982) who showed that, unlike Spinther, Dickinsonia did not develop its discoidal shape from a vermiform juvenile growth stage (Zakrevskaya & Ivantsov, Reference Zakrevskaya and Ivantsov2020).
Dickinsonia was discovered on the Onega Peninsular of the White Sea coast of Russia by M. A. Fedonkin in the summer of 1975 (Keller & Fedonkin, Reference Keller and Fedonkin1976). Better specimens obtained during the next decade suggested that the animal was not precisely bilaterally symmetrical. Rather, its segments alternated across the midline producing what has become known as ‘slide’ or ‘glide’ symmetry (Fig. 4). This is something like the zig-zag axial junctions of the petaloids of Pflug’s erniettomorphs, but was generally interpreted in Russia as offset metamerism rather than mere geometric packing. In 1985, Fedonkin (Reference Fedonkin, Sokolov and Iwanowski1985 b, Reference Fedonkin1998) proposed the new phylum Proarticulata to house Dickinsonia (Class Dipleurozoa), Vendia Keller, Reference Keller and Raaben1969 and related forms. Since then, Andrey Ivantsov and his colleagues have greatly expanded our knowledge and understanding of this proarticulate clade and the putative trace fossils associated with some of its members (Ivantsov, Reference Ivantsov1999, Reference Ivantsov2004, Reference Ivantsov2007, Reference Ivantsov2011, Reference Ivantsov2013; Ivantsov & Malakhovskaya, Reference Ivantsov and Malakhovskaya2002; Ivantsov et al. Reference Ivantsov, Fedonkin, Nagovitsyn and Zakrevskaya2019 a,b,c; Ivantsov et al. Reference Ivantsov, Zakrevskaya, Nagovitsyn, Krasnova, Bobrovskiy and Luzhnaya (Serezhnikova)2020; Ivantsov & Zakrevskaya, Reference Ivantsov and Zakrevskaya2021 a).
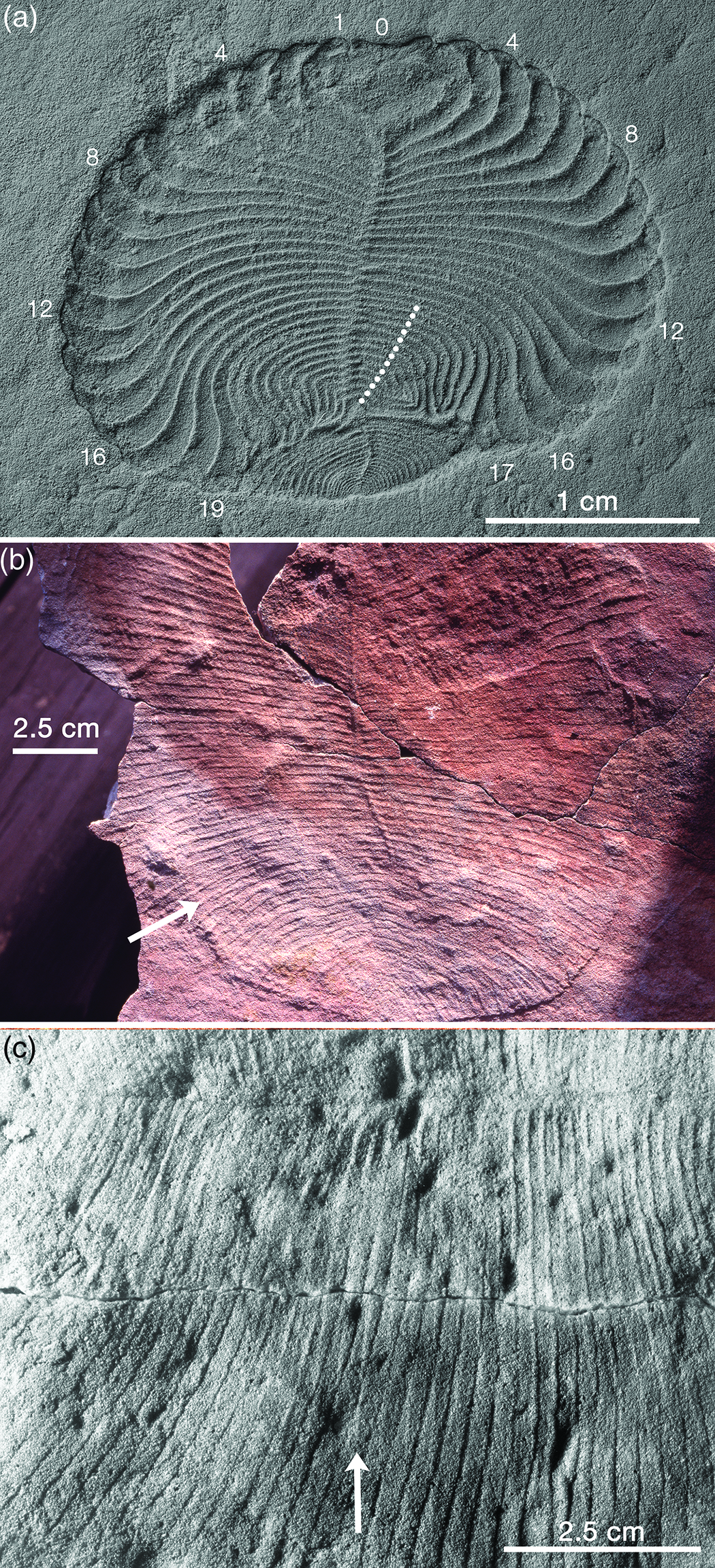
Fig. 4. Anatomical details of Dickinsonia. (a) Beautifully preserved specimen of D. cf. menneri that survived a non-lethal injury and regenerated its tailpiece. The first 17 modules grew normally but the following 13 modules (white dots) were damaged and then grew abnormally (in some cases joining up distally); a new tailpiece was generated from the axis and grew in such a way as to rebuild the oval body outline. Note that although some modules appear to be offset across the midline, others are clearly not offset, and the same number of modules occurs on both sides of the body; RAS PIN 4716/5187, Lyamtsa Formation, Onega Peninsula, Russia, image courtesy of A. Yu. Ivantsov. (b) Unusually large incomplete specimen of D. costata, which displays evidence for both upper and lower surfaces, as shown by the Y-shaped intersections of the module walls (arrow), and it illustrates the ambiguity frequently seen in proarticulates with respect to glide symmetry versus strictly bilateral symmetry; SAM P58615, Ediacara Sandstone Member, Rawnsley Quartzite, Parachilna Gorge. (c) Part of complete specimen of D. costata that also shows both surfaces; arrow points to one of several ridges that reflect one surface of the organism whereas the grooves they cross at a small angle represent the other surface; Sperling & Vinther (Reference Sperling and Vinther2010, fig. S1) identified this specimen as Dickinsonia rex and regarded it as a ‘footprint’, not a body fossil; SAM P58616, Ediacara Sandstone Member, Rawnsley Quartzite, Ediacara, South Australia.
At the time Proarticulata was proposed, similarities in the segmentation of annelids and arthropods were still being used as evidence for their common ancestry (Scholtz, Reference Scholtz2002). Now that it is clear that Articulata (Annelida + Arthropoda) is not a valid group, it is trivially easy to reject the Proarticulata hypothesis (Dunn & Liu, Reference Dunn and Liu2019). However, maybe this is throwing out the baby with the bathwater; a better approach may be to ask whether the repeated isomers/modules/units of the proarticulates share any pattern-forming processes with those controlling segmentation in annelids, arthropoda and chordates. It has become clear that segmentation in those clades evolved convergently (Seaver, Reference Seaver2003; Chipman, Reference Chipman2010; Evans et al. Reference Evans, Droser and Erwin2021). However, seriation of some sort must have a long history in all three main bilaterian clades. For example, early branching panarthropods such as Aysheaia Walcott have stereotypical sets of annulated lobopodial limbs (Chipman & Edgecombe, Reference Chipman and Edgecombe2019), implying that earlier vermiform ecdysozoans had probably already acquired the axial patterning that could be co-opted for generating appropriately spaced legs, as envisaged by Erwin (Reference Erwin2020). These possibilities are explored below.
3. Age and duration of the Ediacara biota
When I entered the University of Queensland early in 1959, Dorothy Hill (1907–1997) began the palaeontology lectures with the then prevalent first approximation that there was no evidence for life before the Cambrian; the preceding ˜3.5 billion years of Earth history were ‘Azoic’. The tide, however, had already turned; Ford’s (Reference Ford1958) article on the Precambrian age of Charnia and Charniodiscus was on its way to Australia by boat, and Glaessner’s (Reference Glaessner1959 b) letter to Nature was about to appear. Furthermore, Arthur Holmes’ (1890–1965) final attempt at a radiometrically calibrated geological timescale, which estimated the base of the Cambrian to be 600 ± 20 Ma, would be published by the end of the year (Holmes, Reference Holmes1959). Fast-forward to the present. The base of the Cambrian is now constrained to be between 538.4 and 538.8 Ma based on recent U–Pb ages from Namibia and Mexico (Linnemann et al. Reference Linnemann, Ovtcharova, Schaltegger, Gärtner, Hautmann, Geyer, Vickers-Rich, Rich, Plessen, Hofmann, Zieger, Krause, Kriesfeld and Smith2019; Hodgin et al. Reference Hodgin, Nelson, Wall, Barrón-Díaz, Webb, Schmitz, Fike, Hagadorn and Smith2020); the oldest Ediacarans postdate the Gaskiers glaciation in American Avalonia at ˜574 Ma (Pu et al. Reference Pu, Bowring, Ramezani, Myrow, Raub, Landing, Mills, Hodgin and Macdonald2016; Matthews et al. Reference Matthews, Liu, Yang, McIlroy, Levell and Condon2021); and the acme of the Ediacaran biota (White Sea assemblage), measured in terms of both diversity and disparity, existed from <558 to >555 Ma.
4. FAQs (frequently asked questions)
4.a. Were the Ediacaran fossils lichens?
Greg Retallack had this ‘annoying idea’ in 1988 and found it surprisingly difficult to falsify (Retallack, Reference Retallack1992, Reference Retallack1994). Judging from the plethora of critiques his numerous articles dealing with this scenario have attracted, others have found it just as hard to accept. Here, we shall limit the discussion to five key elements of the lichen hypothesis: (1) Were the resistant Ediacaran organisms, which are preserved as external moulds on bed bases, woody like plants (Retallack, Reference Retallack1994)? (2) Are the Ediacaran fossils preserved in fossil soils (Retallack, Reference Retallack2012, Reference Retallack2013)? (3) Is the red colour of Australian Ediacaran sediments primary or secondary (Retallack, Reference Retallack2012)? (4) Did the Ediacaran organisms live on land, in the sea or both (Retallack, Reference Retallack2013, Reference Retallack2014)? (5) Were the Ediacaran organisms lichens or fungi (Retallack, Reference Retallack1994, Reference Retallack2007, Reference Retallack2016)?
(1) Woody or not? Wade’s (Reference Wade1968) landmark paper on the preservation of the fossils at Ediacara set the stage for all subsequent work on this topic. She recognized the distinction between resistant animals, which are preserved as external moulds on the bases of beds, and non-resistant ones whose bodies collapsed upon burial. Perceptively, she attributed the formation of counterpart casts to upward movement of relatively incompetent sediment from the underlying bed. This process has been explored experimentally by Bobrovskiy et al. (Reference Bobrovskiy, Krasnova, Ivantsov, Luzhnaya and Brocks2019). We might term it the waterbed hypothesis, one that requires a flexible but inextensible membrane (the mattress), the incompressible fluid it encloses and the bed frame, which holds the mattress in place (Press, Reference Press1978). At Ediacara, these could have been the microbial mat on which the organisms were living, the water-filled pore spaces beneath the mat and the laterally unbounded hydrostatic pressure within the sediment.
The principal alternative is the death mask hypothesis (Gehling, Reference Gehling1999), which requires early mineral cements (iron sulfides or quartz) to support the overlying bed during the decay of the organism (Tarhan et al. Reference Tarhan, Hood, Droser, Gehling and Briggs2016; Liu et al. Reference Liu, McMahon, Matthews, Still and Brasier2019). I prefer the waterbed hypothesis because it relies on conditions that may be regarded as ubiquitous, given the tight association between the fossils and the matgrounds on which they were preserved. Early mineralization fuelled by the decay of organic matter would have had to be remarkably selective to invariably distinguish between resistant and non-resistant organisms, whereas their different resistances to loading should have achieved that automatically. More experimentation is clearly needed. In any case, Retallack (Reference Retallack1994) completely misunderstood the nature of the preservation of the resistant organisms when he compared them with various fossil woods (Waggoner, Reference Waggoner1995). This argument for a lichen affinity is not sustainable.
(2) Are the fossils on terrestrial soils or marine matgrounds? This is a difficult question to address generally so the focus will be on three specific examples. The richly fossiliferous beds at the western edge of the Flinders Ranges (Nilpena, Ediacara, Mt Scott Range) are packages of thin, ripple-marked flaggy sandstones and even thinner intervening sandy ‘shims’ (Fig. 5; Tarhan et al. Reference Tarhan, Droser, Gehling and Dzaugis2017). Retallack (Reference Retallack2019, p. 64) considered these sites to have been ˜60 km inland from the Ediacaran coast and attributed the shims to wind deposition during dry seasons. These clean, sandy quartzites (Fig. 5c) display no textural or chemical evidence for soil formation, so if the Ediacaran organisms were living on these barren braided floodplains, they were doing so in a nutrient-poor environment exposed to enhanced UV under an oxygen-depleted atmosphere (Li et al. Reference Li, Shi, Cheng, Jin and Algeo2020). The arboreomorphs, in particular, may have had a hard time. Yet they persisted, becoming as frequent as ˜60 m−2 in this hostile situation (Droser et al. Reference Droser, Gehling and Jensen2006). Alternatively, if these beds were produced by storm waves in a quiet subtidal setting (Gehling, Reference Gehling2000; Gehling & Droser, Reference Gehling and Droser2013; McMahon et al. Reference McMahon, Liu, Tindal and Kleinhans2021), life for the arboreomorphs should have been easier, as it presumably was beneath the photic zone in Avalonia (Wood et al. Reference Wood, Dalrymple, Narbonne, Gehling and Clapham2003). The extraordinary ecological range implied by identifying the Nilpena flaggy sandstones as fossil soils is one of the best reasons for rejecting the lichen hypothesis.

Fig. 5. Fossiliferous flaggy sandstones at the (a, b) Nilpena National Heritage Ediacaran fossil reserve and (c) Mt Scott Range, western Flinders Ranges, South Australia. Retallack (Reference Retallack2016) considered these kinds of deposits to be palaeosols, formed on a braided stream floodplain ˜60 km inland from the Ediacaran shoreline. The standard view is that they were deposited in a shallow marine environment below fair weather wave base. Overturned bed in (a) is TC-MM3, the lower surface of which (b) is dominated by frond holdfasts (Droser et al. Reference Droser, Gehling, Tarhan, Evans, Hall, Hughes, Hughes, Dzaugis, Dzaugis, Dzaugis and Rice2019). Seen in cross-section (c), these beds bear no resemblance to palaeosols. Coin in (b) and lens cap in (c) are 25 mm and 60 mm in diameter, respectively.
A second specific example is the ‘Muru pedotype’ found beneath a 3–4 cm thick event bed in Bathtub Gorge, central Flinders Ranges, that buried and preserved a death assemblage of Phyllozoon fronds, Aulozoon tubes and Dickinsonia footprints (Gehling & Runnegar, Reference Gehling and Runnegar2021; Retallack, Reference Retallack2013, p. SI4). In this case, the underlying, equally thin sandstone, which is covered with counterpart casts of fossils found on the base of the overlying event bed, is well lithified and easily extracted from the outcrop (Fig. 6). There is no textural or chemical evidence for the A, B and C horizons of the type Muru pedotype (Fig. 6a, b). Suggestions that Phyllozoon was a window lichen and Aulozoon a mycelial rhizome, with Dickinsonia as its mushroom (thallus; Retallack, Reference Retallack2007), are imaginative but unrealistic, given the fact that the putative rhizomes are in the event bed that buried the organisms, not in the underlying ‘palaeosol’ (Fig. 6; Gehling & Runnegar, Reference Gehling and Runnegar2021).

Fig. 6. Fronds of Phyllozoon hanseni and tubes of Aulozoon soliorum on a piece of a 3–4 cm thick event bed from Bathtub Gorge, central Flinders Ranges (Gehling & Runnegar, Reference Gehling and Runnegar2021). The counterpart (a) is the top of the A horizon of a Muru palaeosol according to Retallack (Reference Retallack2013). Both event bed and ‘palaeosol’ are thin, laminated quartz sandstones; the terrestrial palaeosol interpretation is clearly falsified by this example. Arrow marks apparently closed end of one Aulozoon tube. Scale in centimetres. Image courtesy of J. G. Gehling.
The third specific example is from Brachina Gorge, central Flinders Ranges, the type locality for the Wadni and Muru pedotypes (Fig. 7; Retallack, Reference Retallack2012). As we know from political debates, facts are not necessarily enough to overcome deeply held beliefs. In this case, the one example that might help tip the balance is the preservation of Dickinsonia, Parvancorina Glaessner, Reference Glaessner1958 and other taxa on the distended lower surfaces of large load casts at the base of a sandstone bed in Brachina Gorge, central Flinders Ranges (Fig. 7). According to Retallack (Reference Retallack2012), this bed is the C horizon of the type section of his Muru pedotype and was deposited in a sandy river channel before being deformed, not by hydrostatic foundering, but by glacial ice moving over the A horizon loess. This special pleading is necessary to explain sedimentary structures that are otherwise readily attributed to liquefaction or fluidization in aquatic environments (e.g. Owen, Reference Owen1996). Furthermore, the woody properties attributed to Dickinsonia under the lichen hypothesis (Retallack, Reference Retallack1994, Reference Retallack2007) are not expressed in the curvature of a sizeable individual preserved on the convex surface of one rounded load cast (Fig. 7e–g). In each of these examples, the sandstones underlying the fossils have no features of fossil soils and in two of the three cases (Nilpena and Bathtub Gorge) closely resemble sandstones that overlie the fossils. In the third example (Brachina Gorge), the ‘palaeosol’ is a red siltstone that is directly overlain by a thick quartz sandstone that has undergone soft-sediment deformation typical of shallow marine conditions (McMahon et al. Reference McMahon, Liu, Tindal and Kleinhans2021). There is little reason for regarding any of these sediments as terrestrial.

Fig. 7. (d–g) Negative hyporelief casts of Dickinsonia costata on deformed sandstone bed bases, Brachina Gorge, central Flinders Ranges and (a–c) Greg Retallack’s interpretation of these surfaces as the base of the C soil horizon of the type section of the Muru pedotype; after Retallack (Reference Retallack2012, Fig. 14c) © John Wiley and Sons 2012, republished with permission; and Retallack (Reference Retallack2013, fig. S2), reprinted by permission from Springer Nature: Nature, Ediacaran life on land, G. J. Retallack, Copyright (2012). This soft-sediment deformation is attributed to gravitational instability resulting from liquefaction or fluidization (Owen, Reference Owen1996), which is easy to envisage in a shallow marine setting but harder to achieve on a terrestrial floodplain. The convexly curved Dickinsonia in (e) and (f) is on the surface of a load cast, seen in profile in (g); SAM P31895, 60 mm camera lens for scale. The Dickinsonia marked by the arrow in (d) is on the complexly folded bed base shown in profile (left) and in oblique view (right); SAM P34356.
(3) Are the red beds oxidized soils or just outback Australia? Australia’s ‘red centre’ is legendary and the default explanation is regolith chemistry, which has oxidized an ancient landscape that in places may date back to the Mesozoic Era (Twidale, Reference Twidale2016). The difficulty of distinguishing between primary ferric components and those that have been generated by post-depositional processes is exemplified by the saga of the Marble Bar Chert (Rasmussen et al. Reference Rasmussen, Krapež and Muhling2014), where the red colour was for a time used to argue for an oxygen-rich Archaean atmosphere. The red silts in Brachina Gorge, which characterize the type Wadni and Muru pedotypes (Retallack, Reference Retallack2012, Reference Retallack2013), are far more likely to be the products of Cenozoic weathering than Ediacaran soil formation (Pillans, Reference Pillans and Lambers2018).
(4) Were the organisms terrestrial, marine or both? Although Retallack has considered almost every fossiliferous Ediacaran deposit – even Avalonian Newfoundland (Retallack, Reference Retallack2014) – to be no deeper than intertidal, the credibility of his arguments diminishes with distance from South Australia. If the evidence there is equivocal at best, there is little in favour of a terrestrial habitat at any of the classic soft-bodied sites.
(5) Do the fossils themselves resemble lichens or fungi more than animals or plants? This question has been thoroughly explored (Lücking & Nelsen, Reference Lücking, Nelsen, Krings, Harper, Cúneo and Rothwell2018) and the definite answer is ‘no, they do not’. The lichen hypothesis is a false lead that has taken up too much space in the literature.
4.b. Were Ediacaran organisms colonial?
Rangea, Charnia and Charniodiscus automatically became highly individualized colonial animals by Glaessner’s (Reference Glaessner1959 b) promotion of the pennatulacean octocoral hypothesis for these frondose forms from Avalonia, Namibia and Australia. This hypothesis was widely accepted (Jenkins, Reference Jenkins1985; Oliver & Coates, Reference Oliver, Coates, Boardman, Cheetham and Rowell1987) until falsified after almost 50 years by Waggoner & Collins (Reference Waggoner and Collins2002) using a molecular clock, and by Antcliffe & Brasier (Reference Antcliffe and Brasier2006), who contrasted their modes of growth and development. Pennatulacean octocorals are highly evolved cnidarians, well removed from basal groups, so their ability to operate as innervated individuals is a derived feature of the clade. Nevertheless, by attributing this ability to many of the Namibian Petalonamae, Pflug (Reference Pflug1971, Reference Pflug1972 a) saw a transition from frondose taxa such as Rangea and Arborea to animal-like colonies with some of the attributes of echinoderms, arthropods, molluscs and even chordates. These fanciful ideas were based mainly on preservational variants of the three common Namibian genera – Rangea Gürich, 1930, Pteridinium and Ernietta – and have no relevance now other than historical interest. However, Dewel et al. (Reference Dewel, Dewel and McKinney2001) advanced a much more sophisticated and well-received hypothesis along similar lines, using Pteridinium, Charnia, Rangea and Arborea as examples of the progress of colonial duplication and integration during Ediacaran time. In this scheme, the pennatulacean-level integration of Arborea allowed it to operate as a self-sufficient mobile individual and raised the possibility that animals such as Dickinsonia might show how colony integration led to bilaterian segmentation. However, as none of these organisms seems to have been colonial except in the most elementary way (Landing et al. Reference Landing, Antcliffe, Geyer, Kouchinsky, Bowser and Andrease2018), colonialism per se was probably not the evolutionary pathway to more familiar animals (but see Ivantsov, Reference Ivantsov2016 for a different view). Nevertheless, life is built on the LEGO® Principle (McKay, Reference McKay2004). Think elements, biomolecules, ribosomes, cells, organs, segments, individuals, populations, guilds, biomes. In this sense, it is clonal construction rather than colonial construction that characterizes the Ediacaran organisms.
4.c. How and what did Ediacaran organisms eat?
Seilacher (Reference Seilacher1989) had to confront this issue because he could not rely on the mechanisms that were available to those who viewed the Ediacarans as coelenterates, worms and arthropods. He assumed all nutrition needed to be absorbed through the body wall and speculated that the organisms may have depended on endosymbionts for their sustenance (McMenamin, Reference McMenamin1986). He discussed the possibility of photosymbionts for upright taxa in shallow water and chemosymbionts for deepwater taxa and ‘mat recliners’. He even sketched out how endosymbionts in an organism like Dickinsonia could have exploited the oxic–anoxic interface of a matground, obtaining H2S from below and oxygen from seawater. This remains a viable hypothesis for prostrate organisms (Gehling et al. Reference Gehling2005; McIlroy et al. Reference McIlroy, Dufour, Taylor and Nicholls2021). The alternative for an almost sessile creature with no other food-collecting abilities is in situ ventral digestion of the kind used by the living placozoan Trichoplax (Sperling & Vinther, Reference Sperling and Vinther2010).
For the upright fronds and many other forms, all of which have high surface-to-volume ratios, osmotrophy has been the feeding mode of choice (Laflamme et al. Reference Laflamme, Xiao and Kowalewski2009; Ghisalberti et al. Reference Ghisalberti, Gold, Laflamme, Clapham, Narbonne, Summons, Johnston and Jacobs2014). However, Butterfield (Reference Butterfield2020) has made a strong case against having the food absorbed from the outside and instead argued that it was taken in and processed inside, more or less as sponges and cnidarians do. As the food in question is thought to be either dissolved organic carbon (DOC) or particulate organic carbon (POC), I dub these two possibilities the DOC POOL (external feeding) and DOC POC (internal feeding) hypotheses (Fig. 8a). The Devonian rugose corals Heliophyllum and Crepidophyllum, which had digestive epithelium covering their carinate or ‘yard-arm’ septa (Fig. 8b), serve as possible analogues for Butterfield’s DOC POC mode. The ultimate origin of these postulated dispersed and degraded food resources may be provided by evidence from biomarkers (Bobrovskiy et al. Reference Bobrovskiy, Hope, Golubkova and Brocks2020). However, the high density of substrate occupation by some frondose taxa (200–1000 m−2; Ivantsov, Reference Ivantsov2016) raises questions about supply. Another worry is the metabolic cost of keeping sizeable fronds inflated if Butterfield’s (Reference Butterfield2020) DOC POC hypothesis is correct.

Fig. 8. Cartoons aimed at illustrating the high surface to area relationships of (a) epidermal cross-sections of rangeomorphs and (b) Devonian rugose corals. The nested curves in (a) are known as ‘Koch snowflakes’; they are produced by duplications and rotations of a Koch curve (McCartney, Reference McCartney2021). They simulate the increase in complexity with size displayed by rangeomorph fronds during growth (Narbonne, Reference Narbonne2004 b). The green area represents the interior of the largest shape, which might have contained and processed dissolved and particulate carbon (DOC, POC). Under the DOC POOL hypothesis, food is absorbed directly from ocean water (Laflamme et al. Reference Laflamme, Xiao and Kowalewski2009); the DOC POC hypothesis assumes that food was taken in and processed internally (Butterfield, Reference Butterfield2020). (b) Drawings of two transverse sections of the rugose corals (A) Heliophyllum and (C) Crepidophyllum illustrate how second-order ridges on major and minor carinate septa increase the area available for digestion by the gastrodermis (red). The directive axis of Crepidophyllum is indicated by arrows; this key-hole coral is shown in what was standard rugose coral orientation but should be rotated through 180° for comparison with other anthozoans (Oliver, Reference Oliver1980). From Nicholson (Reference Nicholson1878), reproduced with permission from http://www.tandfonline.com.
Another insight into the nutrition of Ediacaran organisms may come from the stunning report of abundant derivatives of cholesterol in coalified cadavers of Dickinsonia at a White Sea locality (Bobrovskiy et al. Reference Bobrovskiy, Hope, Ivantsov, Nettersheim, Hallmann and Brocks2018). Cholesterol is a molecule that stiffens the membranes of eukaryotic cells. It is the dominant sterol in metazoans but is found in lesser amounts in other eukaryotes, most notably red algae (Brocks et al. Reference Brocks, Jarrett, Sirantoine, Hallmann, Hoshino and Liyanage2017). In an adult human, cholesterol forms about 0.33 % of total body weight so the amount present in any Dickinsonia carcass is likely to have been <1 %. In herbivores, all of the cholesterol may be assumed to have been produced in situ, but omnivores and carnivores acquire significant amounts via their diets. Thus, the biomarkers found in Dickinsonia have some potential for understanding both its affiliation and its metabolism. However, any metabolic interpretation is complicated by what happens to the biomolecules following excretion, ingestion, death, burial and diagenesis. In Dickinsonia, the most abundant fossil steroids (steranes) are 5-β cholestane (sometimes known as coprostane) and its monoaromatic equivalents. This is surprising because most cholesterol is converted abiologically into 5-α cholestane, which is more stable and retains the trans stereochemistry of cholesterol and other natural steroids, unlike coprostane. Diagenetic isomerization normally drives the 5-β/5-α ratio towards an equilibrium value of ˜0.65, so ratios as high as 5.5 in the White Sea Dickinsonia compressions require explanation (Bobrovskiy et al. Reference Bobrovskiy, Hope, Ivantsov, Nettersheim, Hallmann and Brocks2018).
In humans and some other animals, anaerobic bacteria can convert cholesterol in the gut into coprostanol – which dehydrates to coprostane – either directly or via intermediates (Kriaa et al. Reference Kriaa, Bourgin, Mkaouar, Jablaoui, Akermi, Soussou, Maguin and Rhimi2019). If this is what caused the elevated 5-β/5-α cholestane ratios in Dickinsonia, how did obligate anaerobes gain access to cholesterol? If Dickinsonia had a gut and digestive system (Ivantsov, Reference Ivantsov2011), cholesterol is unlikely to have been part of the diet as the only environmentally available sterol seems to have been stigmasterol from green algae and unicellular heterotrophs (Bobrovskiy et al. Reference Bobrovskiy, Hope, Ivantsov, Nettersheim, Hallmann and Brocks2018, Reference Bobrovskiy, Hope, Golubkova and Brocks2020). Most of the cholesterol in Dickinsonia must have come from its tissues and it is therefore necessary to implicate anaerobes in the decay process, something not seen in younger forensic, archaeological or palaeontological contexts (Melendez et al. Reference Melendez, Grice and Schwark2013; von der Lühe et al. Reference von Der Lühe, Birk, Dawson, Mayes and Fiedler2018). However, this was Bobrovskiy et al.’s (Reference Bobrovskiy, Hope, Ivantsov, Nettersheim, Hallmann and Brocks2018) preferred explanation for the elevated 5-β/5-α ratios.
Bobrovskiy et al. (Reference Bobrovskiy, Hope, Golubkova and Brocks2020) pointed out that unicellular eukaryotes are a far better food source for early animals than are bacteria and suggested that the availability of high nutritional quality algal biomass may have triggered the Ediacaran radiation of metazoans. The fact that algal stigmasterols were not found in Dickinsonia may perhaps be explained if the unicellular eukaryotes were digested intracellularly rather than being incorporated into an alimentary canal system; shrimp fed on algal sterols excrete them ‘qualitatively and quantitatively’ (Bradshaw et al. Reference Bradshaw, O’Hara, Cornert and Eglinton1990). The same logic could be applied to explain the dearth of bacterial hopanes in Dickinsonia. Hopanes are the carbon skeletons of hopanoids, which some bacteria use instead of steroids to stiffen their cell membranes; the hopane/sterane ratio of the fossils is ˜0.5 (Bobrovskiy et al. Reference Bobrovskiy, Hope, Ivantsov, Nettersheim, Hallmann and Brocks2018, table S3) compared with the enclosing sediments, which have a ratio of ˜3 (Bobrovskiy et al. Reference Bobrovskiy, Hope, Golubkova and Brocks2020, table S1). Thus, it seems that the biomarker evidence supports a lifestyle based on poriferan-style phagocytosis rather than bilaterian extracellular digestion (Steinmetz, Reference Steinmetz2019); the former would destroy membrane molecules cell-by-cell soon after ingestion (see also McIlroy et al. Reference McIlroy, Dufour, Taylor and Nicholls2021).
The other principal hypothesis for vendobiont nutrition, endosymbiosis, may also be examined from the biomarker perspective. If Dickinsonia had been packed full of bacterial chemosymbionts like the trophosome of the vent worm Riftia pachyptila (Jones, Reference Jones1981; Bright & Sorgo, Reference Bright and Sorgo2003), then their presence should be reflected in the hopane/sterane ratio, which seems not to be the case. However, if the endosymbionts were photoautotrophs like coral zooxanthellae, then their potential biosignatures may depend both on their nature and their abundance. Nevertheless, there is no indication from the biomarkers that Dickinsonia housed any kind of endosymbiont. Thus, it is most likely that Dickinsonia was a phagocytic ingester of prokaryotes and/or microscopic eukaryotes. If Bobrovskiy et al. (Reference Bobrovskiy, Hope, Golubkova and Brocks2020) are correct in their supposition that eukaryotes were the principal food source, then there are two possible supply pathways: planktonic green algae (Bobrovskiy et al. Reference Bobrovskiy, Hope, Golubkova and Brocks2020) or benthic members of the meiofauna (Deline et al. Reference Deline, Greenwood, Clark, Puttick, Peterson and Donoghue2018). How either kinds of organisms could be captured and taken in remains a mystery, but placozoan-like grazing on cyanobacterial mats (Sperling & Vinther, Reference Sperling and Vinther2010) is equally difficult to envisage. To paraphrase Ellis Yochelson (1928–2006), Quo vadis Dickinsonia? On the other hand, Ivantsov & Zakrevskaya (Reference Ivantsov and Zakrevskaya2021 b) have made a compelling case for planktotrophy and dorsal ciliary tract feeding in the Trilobozoa.
4.d. Could Ediacaran animals move?
A number of resting and movement traces have been attributed to Ediacaran organisms, but two kinds stand out: (1) overlapping resting traces attributed to movements made by individuals of Dickinsonia and Yorgia Ivantsov, Reference Ivantsov1999; (2) scratch marks (Kimberichnus Ivantsov, Reference Ivantsov2013) that are frequently associated with body fossils of Kimberella Wade, Reference Wade1972 b (Ivantsov, Reference Ivantsov2009, Reference Ivantsov2013; Gehling et al. Reference Gehling, Runnegar and Droser2014). For the purpose of this discussion, I assume that both were produced by the organisms during life rather than being the result of environment-driven transport (McIlroy et al. Reference McIlroy, Brasier and Lang2009), given their taxonomic specificity. So the question is, was this by ciliary gliding (Martin, Reference Martin1978), amoeboid crawling (Bond & Harris, Reference Bond and Harris1988; Arendt et al. Reference Arendt, Benito-Gutierrez, Brunet and Marlow2015; Brunet & King, Reference Brunet and King2017) or muscular motion, the three energetic methods of movement available to animals? My money is on the first for Dickinsonia and Yorgia; for Kimberichnus, it is more important to decide whether it was all or only some of the animal that was moving. We can say ‘animal’ more comfortably here because motility is one feature that helps to distinguish animals from all other kinds of megascopic life.
Living multicellular choanoflagellates use the muscle protein myosin for movement (Brunet et al. Reference Brunet, Larson, Linden, Vermeij, McDonald and King2019). They can invert a cup-shaped ‘colony’ so that the collar cells can face inwards or outwards. And some sponges can move, albeit slowly and inefficiently (Bond & Harris, Reference Bond and Harris1988). If we think of life at the turn of the eon, being able to move was about as valuable a thing as anyone could imagine. Hyoliths, for example, seem to have evolved oar-like calcareous poles (helens) in order to be able to move a little (Runnegar et al. Reference Runnegar, Pojeta, Morris, Taylor, Taylor and McClung1975; Martí Mus et al. Reference Martí Mus, Jeppsson and Malinky2014). Moving first by cellular processes, especially ciliary gliding, could have been the first step towards animal mobility.
If the Dickinsonia and Yorgia footprints are locomotion trails, then it is striking that they are unidirectional in a way that conforms to the traditionally assumed anterior–posterior axis of the body (Glaessner & Wade, Reference Glaessner and Wade1966; Runnegar, Reference Runnegar1982). What was the motive of this unidirectional motion – best shown on the 1T-NA surface at Nilpena as documented by Evans et al. (Reference Evans, Gehling and Droser2019) – and how was it specified? It may be useful to think of minimal requirements such as navigation by solar tracking and photoreceptors no more complicated than flatworm eyespots. Even slime moulds display some phototaxis (Bonner & Lamont, Reference Bonner and Lamont2005).
Although passive transport by bottom currents seems unlikely to have produced the serial footprints of Dickinsonia and Yorgia, there is at least one good example of probable passive transport, the holotype of D. tenuis, which overlies the sand-filled stem of a felled Arborea that retains both the circular holdfast and the lower branches of the frond (Glaessner & Wade, Reference Glaessner and Wade1966, pl. 103, Fig. 1).
4.e. What was the composition of the tough organic integument?
There are two approaches to this question, phylogenetic and taphonomic. If, for example, Dickinsonia was an annelid then its body wall should have been constructed from a chitinous and collagenous cuticle strengthened by circular and longitudinal muscles and connective tissue. Similarly, if Charnia and Charniodiscus were coelenterates, their body walls should have been composed of collagenous mesogloea sandwiched between inner and outer layers of epithelial cells. Alternatively, the preservation of the fossils themselves might suggest that they were made from a leathery (collagenous) material, plant-like biopolymers or something else.
With characteristic perceptiveness, Seilacher (Reference Seilacher1989) described the vendozoan integument as flexible but also malleable, watertight yet permeable, and cuticular but expandable during growth. Noting that the integument of Ernietta was both flexible and elastic, Dzik (Reference Dzik1999) concluded that it must have been composed of collagen and served, like the myosepta of cephalochordates, to enclose the muscle blocks of a hydrostatic skeleton. Although collagenous macromolecular structures are sometimes preserved, most notably in graptolite periderm (Runnegar, Reference Runnegar1986), they are not chemically proteins, which disappear swiftly unless encased in mineral skeletons. So, although we know a good deal about the biochemical components of the membranes of the cells of Dickinsonia (Bobrovskiy et al. Reference Bobrovskiy, Hope, Ivantsov, Nettersheim, Hallmann and Brocks2018), there is as yet no ultrastructural or chemical evidence for the nature of the body wall of any Ediacaran soft-bodied organism.
5. Some comments on key taxa: Pteridinium, Ernietta, Dickinsonia, Arborea, Kimberella
5.a. Pteridinium Gürich, Reference Gürich1933
Pteridinium is an exemplar of the Nama association, in that it is a foliated organism formed of three quilted vanes and is commonly preserved in three dimensions within sandstone beds (Richter, Reference Richter1955; Glaessner & Wade, Reference Glaessner and Wade1966; Pflug, Reference Pflug1970; Jenkins, Reference Jenkins, Lipps and Signor1992; Narbonne et al. Reference Narbonne, Saylor and Grotzinger1997; Dzik, Reference Dzik1999; Meyer et al. Reference Meyer, Elliott, Wood, Polys, Colbert, Maisano, Vickers-Rich, Hall, Hoffman, Schneider and Xiao2014 b). It has been reconstructed in several different ways but its affinities and mode of life remain controversial. One prominent hypothesis – that Pteridinium was canoe-shaped and lived partly or wholly within the sediment in which it is found (Pflug, Reference Pflug1970; Grazhdankin & Seilacher, Reference Grazhdankin and Seilacher2002) – is almost certainly incorrect. It is most likely that Pteridinium was the principal and perhaps propagative part of an upright organism that is nearly always preserved as a transported, deformed and pliable shroud. Its early life stage and attachment structure, if any, may not have been identified. Given its threefold symmetry, it is even possible that Pteridinium is the dispersed frond of one or more coeval trilobozoan discs, such as Tribrachidium Glaessner in Glaessner & Daily, Reference Glaessner and Daily1959 or Rugoconites Glaessner & Wade, Reference Glaessner and Wade1966.
Gürich (Reference Gürich1933) had only two inferior specimens of Pteridinium to work with and did not discover the third vane. Richter (Reference Richter1955) had much better material but came to the strange conclusion that specimens with three vanes were caused by the close packing of left- and right-handed two-vaned individuals, which were twinned like crystals during growth from the sea floor. Nevertheless, Richter did have a clear view of the mode of life and taphonomy of Pteridinium, imagining it to have been rooted in unconsolidated sediment, grown gregariously and, like kelp forests, flexed with the currents and the tides. His choice of a gorgoniid octocoral – the angular sea whip, Pterogorgia anceps – as the closest living analogue was based on its commonly Y-shaped cross-section plus the fact that gorgoniids are readily rooted within soft substrates whereas kelps normally require rocky or stony bottoms. Richter also concluded that the fronds of Pteridinium were tall and tapered slowly from a maximum measured width of 16 cm. The longest vane available to him was 37 cm, comparable to the longest incomplete individual (41.5 cm) reported by Grazhdankin & Seilacher (Reference Grazhdankin and Seilacher2002), so fronds of Pteridinium simplex could have been a sizeable fraction of a metre in height. However, very few specimens of P. simplex show a close approach to the end of a frond (Fig. 9), and as no termination has been reported, it is possible that growth continued for more than a metre.
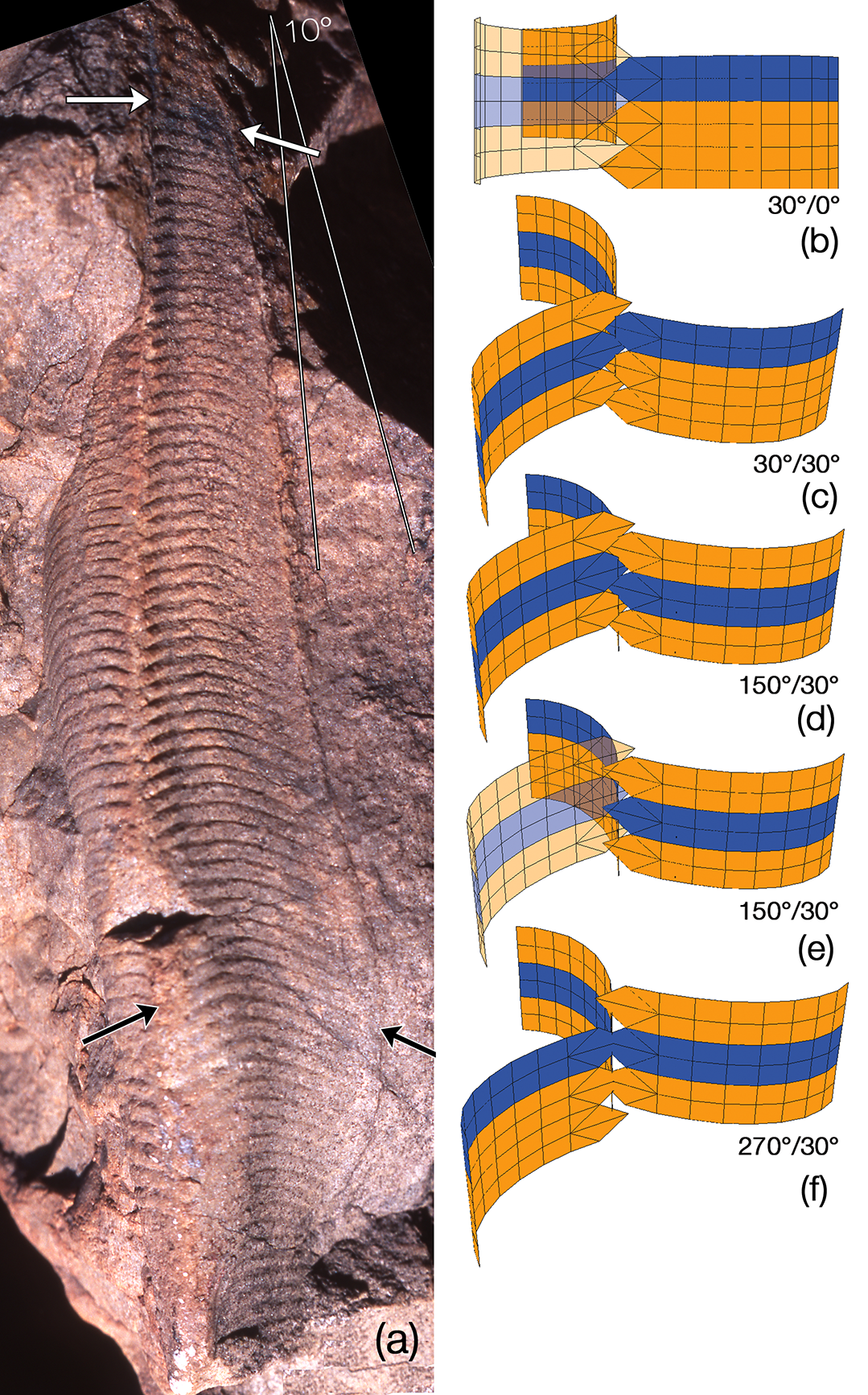
Fig. 9. Geometry of Pteridinium simplex. (a) Exceptional specimen showing tapered approach to the distal end of the organism (top) and measured widths of the right-hand vane between upper arrows (13 mm) and lower arrows (24 mm); unnumbered specimen in the private collection of Wilfried Erni, Plateau Farm, Aus district, Namibia photographed on site in 1996; previously illustrated by Laflamme & Narbonne (Reference Laflamme and Narbonne2008, Fig. 5.5). (b–f) Differently rotated views of part of a model for the orientation and interdigitation of the three vanes. Vanes are modelled as flat curved surfaces set at 120° to each other. Each set of vanes is offset by one module in two of the three intersections (c), (d), (e), but the ends of the modules are opposite each other in the third position (b), (f). Angles are rotations about the axis of the model and tilt with respect to the model. One set of modules is shown in blue for clarity.
The unipolarity of Pteridinium was confirmed by the discovery of additional species, most notably P. carolinaense, famously first described as a trilobite (St Jean, Reference St Jean1973). However, Richter had deduced it from the curvature of the vane quilts, which are convex in the apical direction. The 3D shape of the termination has been less well understood, as two end-member hypotheses illustrate. In the ‘canoe’ model for Pteridinium (Pflug, Reference Pflug1970; Buss & Seilacher, Reference Buss and Seilacher1994; Crimes & Fedonkin, Reference Crimes and Fedonkin1996; Grazhdankin & Seilacher, Reference Grazhdankin and Seilacher2002; Meyer et al. Reference Meyer, Elliott, Schiffbauer, Hall, Hoffman, Schneider, Vickers-Rich and Xiao2014 a; Droser et al. Reference Droser, Tarhan and Gehling2017), two of the vanes form the prows and hull of a canoe-shaped organism that was at least partially buried in the sediment during life; the third vane had the form of a retracted keel running down the length of the canoe. Even more strikingly, Grazhdankin and Seilacher believed that one end of the canoe could reverse direction during extensional growth and that the keel vane of the older, deeper part of the organism could become one of the hull vanes of the younger, shallower section (Grazhdankin & Seilacher, Reference Grazhdankin and Seilacher2002, text-fig. 5C). Additional speculations, not considered here, were interpenetrative growth, where one individual might grow through a pre-existing one without disrupting either, and growth solely within the pore space of the enclosing sand (Crimes & Fedonkin, Reference Crimes and Fedonkin1996).
The alternative end-member model for vane orientation is best illustrated by reconstructions of Swartpuntia germsi (Narbonne et al. Reference Narbonne, Saylor and Grotzinger1997). Although originally described as having four or more vanes, Swartpuntia probably had only three (Narbonne, Reference Narbonne1998, Fig. 1B) and possibly no stalk or holdfast. As such, it is close to Pteridinium, perhaps even congeneric, if you happen to be a lumper rather than a splitter. Because Swartpuntia has always been considered to be an upright organism, its vanes are thought to have been flat and equally spaced radially. The same configuration may be true for Pteridinium, as Jenkins (Reference Jenkins, Lipps and Signor1992) surmised.
The mode of articulation of the quilts of the vanes has also been interpreted in different ways. Ideally, three equally spaced, equal size vanes would be opposite each other across the axis or be offset from each other by an equal amount. In theory, a one third offset of each quilt with respect to the quilts of the adjacent vane, taken in order, would result in [123] or [132] glide symmetries, where 1, 2 and 3 denote the individual vanes (Tojo et al. Reference Tojo, Saito, Kawakami, Ohno, Vickers-Rich and Komarower2007). This was the design adopted by Grazhdankin & Seilacher (Reference Grazhdankin and Seilacher2002, text-fig. 6H) for their canoe keel. However, Pflug (Reference Pflug1970), Jenkins (Reference Jenkins, Lipps and Signor1992) and others have inferred more elaborate architectures, which result in some pairs of vanes being articulated in an elementary zig-zag fashion (Tojo et al. Reference Tojo, Saito, Kawakami, Ohno, Vickers-Rich and Komarower2007, fig. 4A2–A3). In other orientations, there may also be a chain of matrix-filled beads between the proximal edges of the two visible vanes (Grazhdankin & Seilacher, Reference Grazhdankin and Seilacher2002, text-fig. 6F; Pflug, Reference Pflug1970, text-fig. 3E, pl. 21, Fig. 2, text-fig. 8D, pl. 22, Fig. 1). These are the structures that led Jenkins (Reference Jenkins, Lipps and Signor1992) erroneously to reconstruct Pteridinium with two layers of tubular quilts per vane, but they are readily explained as ends of quilts of the third vane, which are visible through apertures in the seam (Figs 9, 10). Thus, Pteridinium did not have perfect three-fold symmetry; rather, it had a best approximation to three-fold symmetry, given the constructional constraints for packing tubular quilts in three dimensions. Achieving the ideal geometry of perfect symmetry (Tojo et al. Reference Tojo, Saito, Kawakami, Ohno, Vickers-Rich and Komarower2007) was probably beyond the developmental capabilities of this organism.

Fig. 10. Toy 3D model of part of a frond of Pteridinium simplex rotated through 120° and 240° to show views of the intersections between adjacent pairs of vanes. Note that in the left and right images the sutures between modules are offset in a zig-zag fashion, whereas in the middle image the modules of adjacent vanes are opposite one another and there is a series of rhomboidal gaps along the axis, as found in a number of fossils.
5.b. Ernietta Pflug, Reference Pflug1966
Ernietta (Fig. 3) was a bag-shaped organism constructed from the same kind of modules as Pteridinium (Pflug, Reference Pflug1970, Reference Pflug1972 b; Jenkins et al. Reference Jenkins, Plummer and Moriarty1981; Dzik, Reference Dzik1999; Elliott et al. Reference Elliott, Trusler, Narbonne, Vickers-Rich, Morton, Hall, Hoffmann and Schneider2016; Ivantsov et al. Reference Ivantsov, Narbonne, Trusler, Greentree and Vickers-Rich2016) and probably Phyllozoon (Gehling & Runnegar, Reference Gehling and Runnegar2021). Reconstructions by Jenkins (in Jenkins et al. Reference Jenkins, Plummer and Moriarty1981) and Ivantsov et al. (Reference Ivantsov, Zakrevskaya and Nagovitsyn2019 c) are remarkably similar and perhaps both incorrect in the same two interconnected ways. It is unequivocal that some specimens from Aar show evidence for walls made of more than one layer of tubular modules (Jenkins et al. Reference Jenkins, Plummer and Moriarty1981; Elliott et al. Reference Elliott, Trusler, Narbonne, Vickers-Rich, Morton, Hall, Hoffmann and Schneider2016) and that many specimens have a constriction at their waist, but I would argue that these two phenomena may be site-specific and causally connected. The constrictions may be due to injury and truncation during a storm surge (Jenkins, Reference Jenkins1985, fig. 1) and the duplicate walls to secondary regrowth of the truncated parts. An analogous situation is seen in cohorts of Dickinsonia menneri from the White Sea (Ivantsov et al. Reference Ivantsov, Zakrevskaya, Nagovitsyn, Krasnova, Bobrovskiy and Luzhnaya (Serezhnikova)2020). If true, this would make Ernietta plateauensis a far simpler organism than previously thought and one that was capable of vegetative regrowth. All known specimens from Nevada show no trace of these features (Smith et al. Reference Smith, Nelson, Tweedt, Zeng and Workman2017). Furthermore, Ernietta from Nevada (Smith et al. Reference Smith, Nelson, Tweedt, Zeng and Workman2017, fig. 3d; Hall et al. Reference Hall, Smith, Tamura, Fakra and Bosak2020, fig. 1b) and at least one specimen from Namibia (Narbonne, Reference Narbonne2004 a, fig. 4b) have modules that terminate distally in pointed tips (Fig. 3a) well separated by open spaces, rather different from the situation seen in the two reconstructions. This is an indication that the modules were independent units that merged during growth rather than subdivisions of the whole (Gehling & Runnegar, Reference Gehling and Runnegar2021). Seilacher (Reference Seilacher1992) had stipulated the opposite when characterizing the Vendobionta.
5.c. Dickinsonia Sprigg, Reference Sprigg1947
The type species, Dickinsonia costata Sprigg, Reference Sprigg1947, is an iconic Ediacaran fossil, a core member of the Vendozoa (Seilacher, Reference Seilacher1989) and a candidate bilaterian animal (Gold et al. Reference Gold, Runnegar, Gehling and Jacobs2015). Seen in plan view as it is presumed to have lived on the sea floor, D. costata is almost circular (Fig. 4b), bilaterally symmetrical and differentiated into a proximal or ‘anterior’ end with a single undivided module lying across the midline and a distal or ‘posterior’ end, from which growth proceeds (Ivantsov et al. Reference Ivantsov, Zakrevskaya, Nagovitsyn, Krasnova, Bobrovskiy and Luzhnaya (Serezhnikova)2020). Suggestions that growth might have proceeded in the opposite direction (Hoekzema et al. Reference Hoekzema, Brasier, Dunn and Liu2017; Dunn et al. Reference Dunn, Liu and Donoghue2018) were falsified recently by the discovery of a cohort of individuals of D. cf. menneri Keller, Reference Keller and Fedonkin1976 in Keller & Fedonkin, Reference Keller and Fedonkin1976, several of which had regenerated the ‘posterior’ end of the body by growing new modules following non-lethal loss (Fig. 4a; Ivantsov et al. Reference Ivantsov, Zakrevskaya, Nagovitsyn, Krasnova, Bobrovskiy and Luzhnaya (Serezhnikova)2020). However, closer inspection of the outline of D. costata reveals that it may be better approximated by the curve known as a cardioid rather than a circle or ellipse, a shape that has not been replicated in any of the proposed growth models. It is also a propensity taken to extremes by Andiva Fedonkin, Reference Fedonkin2002, where the site of ‘terminal addition’ (Gold et al. Reference Gold, Runnegar, Gehling and Jacobs2015) has moved so far forward as to be in the anterior half of the body (Ivantsov, Reference Ivantsov2007, pl. 1, Fig. 6).
As is well known, the body of Dickinsonia costata was constructed from segments, isomers, units or modules, which appeared at an unresolvable size at the ‘posterior’ end and continued to grow and change shape during the life of the animal (Runnegar, Reference Runnegar1982; Evans et al. Reference Evans, Droser and Gehling2017). This alone is a feature of animals rather than plants, kelps or fungi. The modules are defined by raised ridges on the upper surface of specimens preserved in the typical way (concave hyporelief), both in South Australia and Russia, and by grooves in the impressions of lower surfaces (Fig. 4b, c; Seilacher, Reference Seilacher1989, Fig. 5). In large specimens, such as the frequently illustrated D. costata from Brachina Gorge (Wade, Reference Wade1972 a, pl. 5, figs 1, 2; Runnegar, Reference Runnegar1982, figs 4, 5; Seilacher, Reference Seilacher1989, figs 3, 5; Retallack, Reference Retallack2007, Fig. 1A; Budd & Jensen, Reference Budd and Jensen2017, Fig. 4E) or the 1 m sized D. rex Jenkins, Reference Jenkins, Lipps and Signor1992 (Gehling et al. Reference Gehling2005, Fig. 4), the peripheral ends of the ridges are expanded and imbricated in ways that imply that the module walls were stiff like the ‘struts of a glider plane wing’ (Fig. 4a; Seilacher, Reference Seilacher1989, p. 236).
This brings us to the contentious matter of whether or not Dickinsonia exhibits glide symmetry (Fig. 4a, b). The Australian position is ‘no’, based on a large cohort of small individuals of D. costata from a single surface in Crisp Gorge, central Flinders Ranges (Gold et al. Reference Gold, Runnegar, Gehling and Jacobs2015; Reid et al. Reference Reid, García-Bellido, Payne, Runnegar and Gehling2017) as well as numerous specimens from other localities. In contrast, Russian workers identify glide symmetry in all species of Dickinsonia, including D. menneri and D. costata (Ivantsov, Reference Ivantsov2007; Ivantsov et al. Reference Ivantsov, Zakrevskaya, Nagovitsyn, Krasnova, Bobrovskiy and Luzhnaya (Serezhnikova)2020). To some extent, this difference of opinion is hypothesis driven, but it is clear that other members of the same ‘orphan plesion’, the Proarticulata, clearly show this property. Perhaps the most convincing example is the specimen of Andiva ivantsovi Fedonkin, Reference Fedonkin2002 illustrated by Dunn et al. (Reference Dunn, Liu and Donoghue2018, Fig. 4), where the insertion of new modules is shown to have occurred alternately on left and right sides of the body. It is time for Australians to acknowledge that proarticulate glide symmetry is a widespread feature of this putative clade and for Russians to admit that some members of the group do not display it.
Much has been written about the internal anatomy of Dickinsonia, starting with Glaessner and Wade’s discovery of the casts of intestinal caeca on a single specimen of D. costata from Ediacara (Glaessner & Wade, Reference Glaessner and Wade1966, pl. 101, Fig. 4). As confirmation from other specimens failed to materialize, this observation was either disregarded (Runnegar, Reference Runnegar1982) or embellished (Jenkins, Reference Jenkins, Lipps and Signor1992, Fig. 13). Nevertheless, the elevated axial ridge or pair of ridges that are found on many Australian and Russian specimens have been interpreted as evidence for a sediment-filled gut (Wade, Reference Wade1972 a; Runnegar, Reference Runnegar1982; Ivantsov, Reference Ivantsov2004), which terminated at the posterior end of the ‘0 module’ (Fig. 4a; Runnegar, Reference Runnegar1982, Fig. 1C, F; Gehling et al. Reference Gehling2005, Fig. 4a; Sperling & Vinther, Reference Sperling and Vinther2010, Fig. 1A; Gold et al. Reference Gold, Runnegar, Gehling and Jacobs2015, Fig. 1D; Evans et al. Reference Evans, Droser and Gehling2017, Fig. 3b; Hoekzema et al. Reference Hoekzema, Brasier, Dunn and Liu2017, Fig. 1). According to the proarticulate hypothesis, the ‘gut’ consisted of two parallel tubes on either side of an axial partition against which the left and right half modules or ‘isomeres’ abutted (Ivantsov, Reference Ivantsov2011, Fig. 6). The prominence and yet narrowness of this feature in some specimens of Dickinsonia (Gehling et al. Reference Gehling2005, Fig. 4; Ivantsov, Reference Ivantsov2007, pl. 1, Fig. 1), Yorgia (Ivantsov, Reference Ivantsov1999, pl. 1, fig. 4; Evans et al. Reference Evans, Gehling and Droser2019, Fig. 12d) and Andiva (Ivantsov, Reference Ivantsov2007, pl. 1, Fig. 6) make the gut interpretation improbable; a constructional or some other function seems more likely. Particularly telling is the remarkable imprint (Epibaion axiferus) of the putative lower surface of Dickinsonia cf. tenuis (Ivantsov & Malakhovskaya, Reference Ivantsov and Malakhovskaya2002, pl. 2, Fig. 3; Ivantsov, Reference Ivantsov2011, pl. 2, Fig. 1; Ivantsov, Reference Ivantsov2013, pl. 1, Fig. 1), which shows the left and right side modules joined to a well-defined axis. It is hard to imagine how an internal organ could be expressed so crisply externally, although it may have been in a similarly axial position. However, Ivantsov’s (Reference Ivantsov2011) concept of a median septum with longitudinal ‘feeding channels’ on either side makes more sense for the numerous specimens that have elevated ridges on either side of a depressed midline, as is typical of Dickinsonia lissa Wade, Reference Wade1972 a (Runnegar, Reference Runnegar1982, fig. 1A-B; Ivantsov, Reference Ivantsov2007, pl. 1, Fig. 1). Perhaps the axial ridge is nothing more than a topographic high produced during burial by fluid flow from the modules; its prominence in D. lissa may be owing to the fact that the modules are exceptionally narrow and thus constrained all body fluids to move in an axial direction.
Three discoveries made at the White Sea have allowed more elaborate reconstructions to be made of Dickinsonia’s soft parts. First, several specimens of Dickinsonia cf. lissa with complexly corrugated surfaces were discovered at Zimnie Gory (Dzik & Ivantsov, Reference Dzik and Ivantsov2002). The corrugations, which radiate and branch towards the ‘anterior’ direction, were thought to overlie a straight gut and its diverticula as best shown in a cutaway perspective view drawn by Dzik (Reference Dzik2003, Fig. 6). However, it is notable that Dzik’s drawings show no trace of glide symmetry in the internal organs, an anomaly corrected by Ivantsov (Reference Ivantsov2004, Fig. 6); the specimens themselves are ambiguous in this respect. Furthermore, although the corrugations superficially resemble the isomers of other White Sea proarticulates such as Vendia rachiata Ivantsov, Reference Ivantsov2004, it was necessary for Budd & Jensen (Reference Budd and Jensen2017, Fig. 4) to rotate one of the corrugated specimens of D. cf. lissa through 180° to make the visual comparison meaningful. The only other Ediacaran fossil with comparable corrugations is the holotype of Chondroplon bilobatum, which comes from a mass flow sandstone at Ediacara, and thus is preserved in the Nama manner (Wade, Reference Wade1971). Hofmann (Reference Hofmann1988) reinterpreted Chondroplon as a deformed Dickinsonia, a view that was adopted by Dzik & Ivantsov (Reference Dzik and Ivantsov2002). However, it is difficult to exclude the possibility that the corrugations in both taxa are taphonomic effects caused by contractions parallel to the trend of the modules rather than crinkles above incompressible organs (Budd & Jensen, Reference Budd and Jensen2017).
The two other discoveries are feathery and beaded structures within modules of Yorgia and Dickinsonia (Ivantsov, Reference Ivantsov2013, pl. 1, figs 3, 4) and a remarkable incomplete specimen of Dickinsonia cf. tenuis, in which the tubular modules were apparently filled with fine sediment (Ivantsov, Reference Ivantsov2011, pl. 1, Fig. 3). Modules of undescribed specimens of D. rex Jenkins, Reference Jenkins, Lipps and Signor1992 from Nilpena were also filled in this way (J. G. Gehling, pers. comm.). Taken together, these observations tell us little about the internal anatomy of Dickinsonia and the other proarticulates. At best, Seilacher’s characterization of them as fluid-filled ‘pneus’ may serve as the current null hypothesis.
5.d. Arborea Glaessner & Wade, Reference Glaessner and Wade1966
Ford’s chimera of Charnia and Charniodiscus (Fig. 2) serves as a graphical abstract for the Arboreomorpha and Rangeomorpha, two putatively monophyletic clades that may together constitute a monophyletic or paraphyletic group. The tortuous taxonomic trajectory of Arborea reminds us of the obvious similarities yet important differences between the arboreomorphs and rangeomorphs, which have been carefully and thoroughly explored by Canadian and British teams led by Guy Narbonne and Martin Brasier (1947–2014), respectively, over the past several decades.
The first collection of fronds from Ediacara included several slabs of Arborea and one of Charnia. All were referred to Gürich’s genus Rangea, but in an addendum added after Ford’s (Reference Ford1958) article was published, Glaessner noted that one was a Charnia not a Rangea (Glaessner & Daily, Reference Glaessner and Daily1959). By 1960, all of the Ediacaran fronds had become Charnia (Fig. 2; Glaessner, Reference Glaessner1962), but in 1966 Charnia had gone and all of the fronds were now split between two new species of Rangea and the type species of the new genus, Arborea (Glaessner & Wade, Reference Glaessner and Wade1966). The original example of Charnia was rescued by Germs (Germs, Reference Germs1973), who named it Glaessnerina, now a junior synonym of Charnia. Then in 1978, Jenkins & Gehling (Reference Jenkins and Gehling1978) synonymized Arborea with Charniodiscus and she remained in limbo until resurrected from obscurity (Laflamme et al. Reference Laflamme, Gehling and Droser2018; Dunn et al. Reference Dunn, Liu and Gehling2019; Wang et al. Reference Wang, Pang, Chen, Wan, Xiao, Zhou and Yuan2020). All of this equivocation argues for a biological relationship between Charnia and Arborea that is significant at the scale of this review.
The anatomy of Charnia masoni and its relatives have been beautifully revealed by numerous studies of occurrences in Avalonian England and Newfoundland (Narbonne, Reference Narbonne2004 b; Antcliffe & Brasier, Reference Antcliffe and Brasier2006; Gehling & Narbonne, Reference Gehling and Narbonne2007; Laflamme et al. Reference Laflamme, Narbonne, Greentree, Anderson, Vickers-Rich and Komarower2007, Reference Laflamme, Flude and Narbonne2012; Hofmann et al. Reference Hofmann, O’Brien and King2008; Narbonne et al. Reference Narbonne, Laflamme, Greentree and Trusler2009; Brasier et al. Reference Brasier, Antcliffe and Liu2012; McIlroy et al. Reference McIlroy, Hawco, McKean, Nicholls, Pasinetti and Taylor2020). Rangeomorphs are frondose taxa that maximized surface area via fractal growth (Fig. 8). The most obvious links to the Arboreomorpha are their similar body forms and discoidal holdfasts, at least in some members of each group. I treat them as a paraphyletic grade for the sake of simplicity (Fig. 12); cladistic analyses have recovered the Erniettomorpha as sister of the Rangeomorpha (Dececchi et al. Reference Dececchi, Narbonne, Greentree and Laflamme2017; Hoyal Cuthill & Han, Reference Hoyal Cuthill and Han2018), and it is plausible to refer all three groups to Pflug’s Petalonamae. However, major differences in construction of the three groups, summarized in the first five characters of Dececchi et al.’s data matrix (modular or not, branching or not, tubular modules or not, fractal construction or not, differentiated elements or not), also permit the following relationship: (Erniettomorpha (Arboreomorpha, Rangeomorpha)).
5.e. Kimberella Wade, Reference Wade1972 b
When Glaessner & Wade (Reference Glaessner and Wade1966) first described Kimberella (as Kimberia quadrata), Sprigg’s jellyfish hypothesis was state of the art, and so they doubled the visible bilateral symmetry on the assumption that they were looking at a crushed hydrozoan or cubozoan medusa. Wade (Reference Wade1972 b) extended this way of thinking but was unwilling to refer Kimberella to either the Hydrozoa or the Cubozoa, preferring to regard it as something like a stem member of one or both of these groups (Glaessner, Reference Glaessner1984, Fig. 3.2). However, Jenkins (Reference Jenkins1984) confidently considered Kimberella to be ancestral to the living box jellyfish and even speculated that cubozoans refined and perfected their potent venom over an eon of evolution. One reason this jellyfish hypothesis seemed to work so well is that almost every Australian specimen of Kimberella has a well-rounded and clearly formed end (the bell) and fades out at the other end into what may be thought of as tentacles.
The breakthrough with Kimberella came with the discovery of much better material in Russia and its analysis by Fedonkin & Waggoner (Reference Fedonkin and Waggoner1997). Unfortunately, although the bilateral symmetry seems clear, there was and still is no other character that could identify Kimberella as a mollusc or even a bilaterian animal. However, sets of paired scratch marks, found in fan-shaped arrays on bed bases and interpreted as possible arthropod scratch marks, had been previously illustrated by Gehling (Reference Gehling1991, pl. 6, Fig. 3) and their producer adventurously reconstructed by Jenkins (Reference Jenkins, Lipps and Signor1992, Fig. 10). The association of these trace fossils, now known as Kimberichnus teruzzi (Ivantsov, Reference Ivantsov2013; Gehling et al. Reference Gehling, Runnegar and Droser2014), has firmed up the idea that Kimberella was a bilaterian metazoan (Fedonkin et al. Reference Fedonkin, Simonetta, Ivantsov, Vickers-Rich and Komarower2007; Ivantsov, Reference Ivantsov2009, Reference Ivantsov2013).
There are, however, some difficulties with attributing the traces to Kimberella. The body fossils obviously pre-date the deposition of the event bed that buried them, but the time of formation of the traces is more uncertain. Given their similarity to Monomorphichnus Crimes, Reference Crimes1970 (Jenkins, Reference Jenkins, Lipps and Signor1992, Reference Jenkins1995) – one of Seilacher’s (Reference Seilacher2007) trilobite ‘deep undertraces’—they could have been made after the storm sand was deposited. That could explain how Kimberichnus was superimposed on an Aspidella holdfast (Ivantsov et al. Reference Ivantsov, Zakrevskaya, Nagovitsyn, Krasnova, Bobrovskiy and Luzhnaya (Serezhnikova)2020, Fig. 1) and a Phyllozoon frond (Gehling & Runnegar, Reference Gehling and Runnegar2021, fig. S3a); undertraces are constructed with the soft sand in place. The alternative hypothesis, that the traces were made by mining an exposed mat surface, requires the paired scratches to remain open for extended periods of time (e.g. Gehling et al. Reference Gehling, Runnegar and Droser2014, Fig. 6), a problem perhaps solved by Budd & Jensen (Reference Budd and Jensen2017, p. 458), who concluded that the sharpness of the scratches shows ‘that they were not formed in the mat but rather in the sediment underlying the mat.’ However, this is unlikely to be true for the Kimberichnus found in Bathtub Gorge, South Australia, where the underlying bed has a coarse sugary top that contrasts with the fine base of the overlying event bed (Gehling & Runnegar, Reference Gehling and Runnegar2021). The production of Kimberichnus needs additional investigation.
The most striking feature of the anatomy of Kimberella is the so-called ‘crenellated zone’, which encircles most of the body and generally lies inboard of a well-defined rim (Fedonkin & Waggoner, Reference Fedonkin and Waggoner1997). The preservation of this feature varies greatly from non-existent to ladder-like in expanded specimens. It has been reconstructed as a ruff-like extensible mantle (Fedonkin & Waggoner, Reference Fedonkin and Waggoner1997, Fig. 2b; Fedonkin et al. Reference Fedonkin, Simonetta, Ivantsov, Vickers-Rich and Komarower2007, fig. 23d), as the scalloped zone of a dorsal carapace (Ivantsov, Reference Ivantsov2013, Reference Ivantsov2017) and in the box jellyfish phase, as pouched gonads attached to radial canals (Wade, Reference Wade1972 b, text-fig. 6; Jenkins, Reference Jenkins1984, text-fig. 2). The evidence for this crenellated zone being suspended above the sides of the body is slight and in many specimens, small and large, the crenellations extend to the rim. However, in those cases they look more like regularly arranged anticlinal ridges than ruff-like folds. Similar angular ridges are seen in specimens of Temnoxa molliuscula and Keretsa brutoni (Ivantsov, Reference Ivantsov2017; Ivantsov & Zakrevskaya, Reference Ivantsov and Zakrevskaya2021 c), which like Kimberella, have a rounded larger terminus and a tent-like shape following compaction. Australian specimens of Keretsa and Kimberella are even more similar in these respects (Gehling, Reference Gehling2005, fig. 12K; Gehling et al. Reference Gehling2005, Fig. 12).
There are many other well-characterized aspects of the anatomy of Kimberella, but it is the flat and probably muscular base that most obviously suggests an affinity with the Mollusca. However, extended specimens that are connected to fan-shaped arrays of Kimberichnus plausibly made by them, suggest alternative possibilities (Ivantsov, Reference Ivantsov2009; Gehling et al. Reference Gehling, Runnegar and Droser2014), one of which might be an animal of cnidarian grade. It is possible to regard Kimberella as some kind of foraging anemone, anchored by an aboral pedal disc and collecting food with a cuticularized oral apparatus that acted more like a rake than a pair of claws (Ivantsov, Reference Ivantsov2009). It may not have moved around much, if at all, operating more like a strip-mining dragline than a mechanical excavator (Gehling et al. Reference Gehling, Runnegar and Droser2014, Fig. 9).
6. Speculative discussion
6.a. Introduction
Despite all of the research that has been accomplished over the past three quarters of a century and the dedicated contributions of many talented palaeobiologists, the presumed affinities of Ediacaran organisms have, on average, hovered continuously around the coelenterate grade, although there has been significant stemward slippage in recent years (Table 1). The only really positive assignments, such as Glaessner’s Pennatulacea hypothesis, have been falsified, and the current approach of seeking ever more detailed and quantifiable knowledge (Laflamme et al. Reference Laflamme, Narbonne and Anderson2004; Evans et al. Reference Evans, Droser and Gehling2017; Hoekzema et al. Reference Hoekzema, Brasier, Dunn and Liu2017; Hoyal Cuthill & Han, Reference Hoyal Cuthill and Han2018) has not, as yet, led to startling outcomes. Perhaps the time is ripe for some speculative, even outlandish thinking. It may also be useful to review briefly previous ways of dealing with problematical fossils.
Table 1. Chronology of taxonomic assignments to core members of the Ediacara biota. Note the stemward movement as time progresses. Cnidaria highlighted in grey

TG – total group; TSAR – Telonemia + Stramenopila + Alveolata + Rhizaria.
6.b. Prior Problematica
Palaeontologists have long been perplexed by problematical fossils, particularly by those with no obvious living counterparts (Bengtson, Reference Bengtson, Hoffman and Nitecki1986). Famous examples include rudist bivalves (Skelton, Reference Skelton2018), graptolites (Mitchell et al. Reference Mitchell, Melchin, Cameron and Maletz2013), archaeocyaths (Rowland, Reference Rowland2001) and the phosphatic microfossil Microdictyon, which as the authors who named it noted, is ‘shaped like a little net’ (Bengtson et al. Reference Bengtson, Matthews, Missarzhevsky, Hoffman and Nitecki1986). Of these conundrums, rudists were solved first by S. P Woodward, author of A Manual of the Mollusca (1851–1854), in a remarkably advanced article published even before brachiopods were removed from the phylum. Woodward’s words resonate today despite his firm belief in Divine creation rather than Darwinian transmutation: ‘In searching out the affinities of a problematic fossil shell, it is desirable to inquire, first, whether any similar, but less abnormal, forms occur in the same stratum with it, or in formations immediately older or newer. … We think it may be shown, that, by a complete series of cognate forms, the Cretaceous Hippurites are connected with the Oolitic Dicerata and the Tertiary Chamæ.’ (Woodward, Reference Woodward1855, p. 46). Thus, these strange coral-like bivalves (Fig. 11b) were confidently identified as a major extinct clade of the Bivalvia by the mid-nineteenth century (Skelton, Reference Skelton2018; Rineau et al. Reference Rineau, Masse and Villier2020).

Fig. 11. Previously puzzling Problematica. (a) 3D preservation of Ordovician graptolite Amplexograptus maxwelli, Bromide Formation, Oklahoma, showing half-ring construction of periderm (arrow). (b) Coral-like Cretaceous rudist bivalve Barrettia monilifera, Florida Formation, Puerto Rico. (c) Phosphatic shoulder pad of Cambrian lobopod Microdictyon cf. robisoni, Gowers Formation, Queensland. (d) Transverse thin-section of Cambrian regular archaeocyath Loculicyathus alternus, Ajax Limestone, South Australia.
Clarification of the affinities of graptolites followed another path. Roman Kozlowski’s pre-Second World War discovery of extractable, three-dimensionally preserved colonies in Polish Ordovician limestones revealed the fine structure of the periderm to be formed of ‘half rings’ as in living pterobranchs (Fig. 11a; Kozlowski, Reference Kozlowski1947). The hemichordate hypothesis received a second boost when Towe & Urbanek (Reference Towe and Urbanek1972) provided ultrastructural evidence that the graptolite periderm was composed of collagen rather than cellulose or chitin (Runnegar, Reference Runnegar1986), and has been accepted ever since. In this case, it was new information from both the fossils and their living relatives that turned the tide.
Archaeocyaths (Fig. 11d) are another previously problematical group that was passed around phylogenetically speaking until the emergence of two mid-twentieth century hypotheses: an extinct phylum, the Archaeocyatha (Hill, Reference Hill1964) or kingdom, the Archaeta (Zuhuravleva & Myagkova, Reference Zhuravleva and Myagkova1972); or an extinct sponge clade and grade. The second alternative is now mainstream; its acceptance required the SCUBA-enabled discovery of living aspiculate sclerosponges as analogues for archaeocyaths (Vacelet, Reference Vacelet, Conway Morris, George, Gibson and Platt1985; Kruse, Reference Kruse, Jago and Moore1990; Rowland, Reference Rowland2001).
In contrast, Microdictyon (Fig. 11c) was one of the many small shelly fossils that fell out of early Cambrian carbonates dissolved slowly and laboriously in acetic acid (Bengtson et al. Reference Bengtson, Matthews, Missarzhevsky, Hoffman and Nitecki1986). Speculations about its affinity and function were wide ranging and numerous, but the solution seemed absurd when it suddenly appeared. Stefan Bengtson and I were sitting together in a meeting at UCLA when he opened a letter from China that contained a photograph of the Microdictyon animal from Chenjiang. We both began to laugh; no one could have imagined that Microdictyon was the shoulder pads of a lobopodian relative of Conway Morris’s Hallucigenia. Some answers require new evidence rather than imagination.
Applying these lessons to the Ediacaran fossils may help find their place in the tree of life. Here are other guidelines, largely adapted from Dunn & Liu (Reference Dunn and Liu2019): (1) Know that all fossils must be connected to some branch of the extant tree of life; they will either be stem or crown members of their respective clades. (2) Work with the best current consensus tree based on phylogenetic information obtained from living organisms. (3) Assume that most early problematical fossils – orphan plesions – will be stem rather than crown members of their respective clades. (4) Avoid terminology that incorporates a preconceived worldview when naming clades. (5) Use data obtained from populations of individuals rather than individual examples whenever possible.
6.c. Phylogenetics
For a phylogenetic framework, I assume that a monophyletic Porifera rather than Ctenophora is the basal branch of the metazoan tree and that xenocoelomorphs are simplified relatives of echinoderms and chordates (Kapli & Telford, Reference Kapli and Telford2020). The latter assumption leaves a clean Bilateria of the form (Deuterostomia (Ecdysozoa, Lophotrochozoa)), which is ample for this discussion. I also accept tentatively (Fig. 12) the sibling relationship between Cnidaria and Ctenophora proposed by Zhao et al. (Reference Zhao, Vinther, Parry, Wei, Green, Pisani, Hou, Edgecombe and Cong2019) based on their careful analysis of putative Cambrian stem members of the Ctenophora; this resurrects a monophyletic Coelenterata for the two phyla, a position adopted by Cavalier-Smith (Reference Cavalier-Smith2017) for entirely different reasons. The evolution of the metazoan stem lineage has been well reviewed by Cavalier-Smith (Reference Cavalier-Smith2017), Brunet & King (Reference Brunet and King2017) and Budd & Jensen (Reference Budd and Jensen2017); there is nothing to add except to note that Apoikozoa of Budd and Jensen (Choanoflagellata + Metazoa) yields to Choanozoa of Brunet and King.

Fig. 12. Best-guess scenario for the relationships of Ediacaran organisms discussed in this review at the time of the Proterozoic–Phanerozoic boundary, when all of the groups shown in blue could have been easily accommodated in Seilacher’s ‘Vendozoa’. Ichthyosporea and Filasterea are omitted but lie between Fungi and Choanoflagellata; for the Metazoa, Porifera is treated as both basal and monophyletic, Placozoa (not shown) probably falls somewhere between sponges and coelenterates and Coelenterata consists of the sister phyla Cnidaria and Ctenophora, which may be either a clade (line) or a grade (shading). Ernietta is highlighted because it illustrates the degree of complexity that may have led to the early diverging eumetazoan phyla. The position of the Proarticulata is uncertain as it depends on whether or not its members had an alimentary canal (blind or through gut). If not, they should be lower in the diagram. K? stands for Kimberella, which as explained in the text, may have been a kind of foraging coelenterate; PDA is the protostome–deuterostome ancestor.
There is a continuing consensus that rangeomorphs and arboreomorphs are of cnidarian grade (Table 1), being constructed from inner and outer layers of cells with a collagenous structural layer of some sort in between. Cavalier-Smith (Reference Cavalier-Smith2017) suggested that this architecture could be pre-sponge, formed of layers of choanocytes on all unattached surfaces, but Dufour & McIlroy (Reference Dufour, McIlroy, Brasier, McIlroy and McLoughlin2017, Reference Dufour and McIlroy2018) thought this unlikely and instead proposed a pre-placozoan alternative with phagocytic cells on surfaces that are not exposed to ocean water. Similar ideas were foreshadowed by Pflug (Reference Pflug1972 a). Given the architectural complexity of rangeomorphs and arboreomorphs in comparison with sponges, including the Archaeocyatha, a pre-placozoan or pre-cnidarian grade seems more likely. As a starting point, rangeomorphs and arboreomorphs are placed somewhere between sponges and coelenterates on the metazoan tree (Fig. 12). Budd & Jensen (Reference Budd and Jensen2017) arrived at a similar conclusion but put rangeomorphs beneath the Porifera.
That is the easy part. But is it possible to use any of the other core Ediacarans to show how more complicated animals might have evolved? Glaessner and Wade apparently thought not: ‘The Ediacara fauna in general appears too young to be the repository of links between many phyla. Coelenterates, annelids and arthropods were quite diversified by that time (Glaessner Reference Glaessner1971)’ (Wade, Reference Wade1972 a, p. 189). This was prior to the use of molecular clocks to estimate divergence times (e.g. Dohrmann & Wörheide, Reference Dohrmann and Wörheide2017), but given the fact that stem ecdysozoans must date from at least the time of the first appearance datum (FAD) of Treptichnus pedum Seilacher, Reference Seilacher, Schindewolf and Seilacher1955 (Kesidis et al. Reference Kesidis, Slater, Jensen and Budd2019) and that the terminal Ediacaran worm Sabellidites cambriensis Yanishevsky, Reference Yanishevsky1926 may be a crown group annelid (Moczydłowska et al. Reference Moczydłowska, Westall and Foucher2014; but see Georgieva et al. Reference Georgieva, Little Crispin, Watson, Sephton, Ball and Glover2019), the same concern may still apply. On the other hand, Zhao et al. (Reference Zhao, Vinther, Parry, Wei, Green, Pisani, Hou, Edgecombe and Cong2019) appear to have demonstrated considerable evolution of body form within the Cambrian in animals of coelenterate grade, so perhaps the game was not over by the later Ediacaran.
Two transformations in animal body plan that may be detectable in Ediacaran biology are the appearances of the cnidarian coelenteron and the bilaterian gut. These are traditionally attributed to larval innovations, as elegantly summarized in verse by Walter Garstang (Reference Garstang1966). However, even though Haeckel’s (Reference Haeckel1874) gastraea theory was derived from a sponge gastrula, the atrium (spongocoel) of sponges is not likely to be homologous with the coelenteron of cnidarians (Nielsen, Reference Nielsen2008). Cavalier-Smith (Reference Cavalier-Smith2017) disagreed, arguing that the inside of a simple asconoid sponge is the forerunner of the cnidarian coelenteron. To some extent, this proposal resembles Pflug’s petaloid cavity or ‘centrarium’ in that an enclosed piece of the open environment is co-opted for a body cavity. Cavalier-Smith then took the extraordinary step of transforming the cnidarian coelenteron into the bilaterian coelom by extending the pharynx into a gut that pierced the aboral body wall, creating an anus (Cavalier-Smith, Reference Cavalier-Smith2017, Fig. 3).
There may be another pathway to the cnidarian coelenteron that bypasses sponges completely. Imagine Ernietta as a stem cnidarian with the modules filled with mesenchyme, as envisaged by Dufour & McIlroy (Reference Dufour, McIlroy, Brasier, McIlroy and McLoughlin2017), and with seams between modules that will eventually become the mesenteries of an anthozoan polyp. The tapered growing ends of the modules may then have acquired nematocysts and became more muscular, allowing the animal to begin to collect higher quality food than previously possible. Furthermore, the bipolar symmetry of Ernietta may be superficial, given its close resemblance to Pteridinium and to a lesser extent Phyllozoon (Figs 3, 6; Gehling & Runnegar, Reference Gehling and Runnegar2021). If so, cnidarians may have inherited ‘bilaterian’ body axes (L–R, A–P, D–V) from erniettomorphs.
Phylogenomic studies support a monophyletic Cnidaria comprising two principal clades, Anthozoa and Medusozoa (Hydrozoa + (Staurozoa, Scyphozoa, Cubozoa)), and characterize the ancestral cnidarian as a solitary, non-symbiotic polyp that propagated by means of a planula larva (Pratlong et al. Reference Pratlong, Rancurel, Pontarotti and Aurelle2017; Kayal et al. Reference Kayal, Bentlage, Sabrina Pankey, Ohdera, Medina, Plachetzki, Collins and Ryan2018; Khalturin et al. Reference Khalturin, Shinzato, Khalturina, Hamada, Fujie, Koyanagi, Kanda, Goto, Anton-Erxleben, Toyokawa, Toshino and Satoh2019). Although (Khalturin et al. Reference Khalturin, Shinzato, Khalturina, Hamada, Fujie, Koyanagi, Kanda, Goto, Anton-Erxleben, Toyokawa, Toshino and Satoh2019) felt that the genetic and morphologic differences between anthozoan and medusozoan body forms were too great to support anything more complicated than a planula as their mutual last common ancestor, the presence of conserved minicollagen gene clusters, the products of which encode the principal structural protein of nematocytes in all major cnidarian clades, is an indication that the ancestor must have been large enough to need and use nematocysts. This argues for extreme divergence of current polyp architecture rather than a larval level latest common ancestor (LCA).
All anthozoans, including Palaeozoic rugose corals (Fig. 8b), are bilaterally symmetrical (Oliver, Reference Oliver1980). In the model anthozoan, the starlet sea anemone Nematostella Stephenson, Reference Stephenson1935 (Frank & Bleakney, Reference Frank and Bleakney1976), the bilateral symmetry is expressed first in the early planula larva by an elongation of the oral opening and then by the disposition of the first eight mesenteries and the formation of the first four tentacles, two on either side of the symmetry plane (He et al. Reference He, Del Viso, Chen, Ikmi, Kroesen and Gibson2018). Subsequently, one end of the oral aperture becomes enlarged to form a ciliated groove (siphonoglyph), which serves as a landmark during further development. Thus, Nematostella and other anthozoans have a vectorial ‘directive axis’ that lies in the plane of symmetry (Fig. 8b). This axis expresses the products of some Hox and other positional genes during development and is therefore equated with the anterior–posterior axis of bilaterians (He et al. Reference He, Del Viso, Chen, Ikmi, Kroesen and Gibson2018; Nielsen et al. Reference Nielsen, Brunet and Arendt2018; Technau & Genikhovich, Reference Technau and Genikhovich2018). It follows that the oral–aboral axis of cnidarians is equivalent to the ventral–dorsal axis of bilaterians and perhaps to the lower and upper surfaces of Trichoplax, respectively (DuBuc et al. Reference DuBuc, Ryan and Martindale2019). This 3D Cartesian coordinate system pre-dates at least the coelenterates in metazoan evolution and may have been present in classic Ediacarans, including all of the taxa reviewed here. If so, how can this help us understand Ediacaran palaeobiology?
The two principal proposals for the derivation of the bilaterians from coelenterates are Haeckel’s (Reference Haeckel1874) gastrea hypothesis and von Graff’s paedomorphic planula hypothesis (Hejnol & Martindale, Reference Hejnol, Martindale, Telford and Littlewood2009). Each requires larval adaptations that are expressed as new kinds of adults. In Haeckel’s case, the archenteron of the larva, which resulted from gastrulation, becomes the gut of the adult. In protostomes, the oral aperture of the larva becomes the mouth, whereas in deuterostomes it becomes the anus. This problem of initial order was overcome by Sedgwick’s (Reference Sedgwick1884) amphistomy hypothesis, which derived the mouth and anus from opposite ends of an elongate blastopore; loss of one or other larval apertures then accounted for the protostome–deuterostome division (Nielsen, Reference Nielsen2008; Hejnol & Martindale, Reference Hejnol, Martindale, Telford and Littlewood2009). Nielsen et al. (Reference Nielsen, Brunet and Arendt2018) have advanced a strong case for early amphistomy, whereas Hejnol & Martindale (Reference Hejnol, Martindale, Telford and Littlewood2009) have equally firmly rejected both amphistomic gastrulation and Haeckel’s gastrea. Nevertheless, amphistomic gastrulation helps solve the topological problem of deriving the A–P axis of a bilaterian from the directive axis, rather than the oral–aboral axis, of a coelenterate.
For the Ediacaran White Sea biota, these matters were investigated by Fedonkin (Reference Fedonkin1985 a,b) using the principles of promorphology (animal symmetry) expounded by Beklemishev (Reference Beklemishev1969). However, in contrast to Sedgwick (Reference Sedgwick1884), who suggested that the bilateral symmetry of an annelid is inherited from amphistomic development in the directive plane of an anemone, Fedonkin thought that the Ediacaran ‘medusoids’ were jellyfish of various symmetry classes (infinite, infinite and radial, uncertain, three-fold, four-fold, etc.) that led directly to the more elongated but still somewhat radially arranged Proarticulata (Dickinsonia et al.). Thus, the glide symmetry of proarticulates represented an early attempt to transform radial elements into metameres, and the anterior–posterior axis of bilaterians was developed during this process. A similarly vague derivation was proposed by Malakhov (Reference Malakhov2016, p. 295), who speculated that ‘it was the mobile mode of life [of Ediacaran proarticulates] that determined the development of antero-posterior polarity and bilaterial symmetry in the common ancestors of Cnidaria and triploblastic Bilateria.’ This scenario is more clearly described by Arendt et al. (Reference Arendt, Benito-Gutierrez, Brunet and Marlow2015), who treated the ‘gastric pouches’ of Dickinsonia (Dzik, Reference Dzik2003, Fig. 8; sediment-filled modules, as discussed in Section 5.c) and the compartments defined by the four pairs of mesenteries of a Nematostella ‘edwardsia larva’ (Daly, Reference Daly2002), as homologous structures. They also suggested that the terminal addition of modules in Dickinsonia (Runnegar, Reference Runnegar1982; Gold et al. Reference Gold, Runnegar, Gehling and Jacobs2015; Ivantsov et al. Reference Ivantsov, Zakrevskaya, Nagovitsyn, Krasnova, Bobrovskiy and Luzhnaya (Serezhnikova)2020) and mesenteries in anthozoans have a common developmental origin. The implication here is that the vagile habits of proarticulates are responsible for the directive axis in cnidarians, which is the opposite of what Sedgwick thought.
On the other hand, it may be that the quasi-bilateral symmetry of rangeomorphs, arboreomorphs, some erniettomorphs, and dickinsoniomorphs and their growth by terminal addition sensu lato formed the basis, first for coelenterate and then bilaterian symmetry, following Sedgwick’s mechanism. In this case the holdfasts of fronds, the proximal ends of erniettomorphs and the ‘head’ regions of dickinsoniomorphs are anatomically ‘anterior’ in the bilaterian sense and their growing tips are ‘posterior’. The coelenteron of cnidarians is derived from the ‘centrarium’ of an Ernietta-like animal, as previously explained, and evolutionary amphistomy (Nielsen et al. Reference Nielsen, Brunet and Arendt2018), or perhaps evolutionary deuterostomy (Cavalier-Smith, Reference Cavalier-Smith2017; Steinmetz et al. Reference Steinmetz, Aman, Kraus and Technau2017; Nielsen et al. Reference Nielsen, Brunet and Arendt2018; Steinmetz, Reference Steinmetz2019), then leads to the Bilateria. It is a wild speculation but one that may help focus thinking on the path ahead. The phylogenetic implications of this suggestion are illustrated in Figure 12. According to this theory, those forms with apparently bipolar symmetry, such as Fractofusus and Ernietta, are secondarily bipolar, having been able to relax the directive axis developmental constraints for lifestyle reasons. Glide symmetry represents a transitional step between the zig-zag axes of rangeomorphs, arboreomorphs, erniettomorphs and proarticulates and the sagittal plane symmetry of the Bilateria. Proarticulates may or may not have had a bilaterian gut, so their phylogenetic position remains uncertain (Fig. 12). Kimberella and other Ediacaran taxa not treated here are wildcards that are not easy to place, but there is little evidence to suggest that they were higher in the metazoan tree than coelenterate grade.
7. Envoi
As Breandán MacGabhann (Reference MacGabhann2014) has aggressively noted, there is no such thing as the Ediacara biota; many other complex organisms were around at the same time. Nevertheless, to co-opt an ancient cliché, ‘we recognize it when we see it’. It is in that spirit that we continue to explore the biology and phylogenetic meaning of these fascinating creatures.
8. Methods
The Koch snowflakes (Fig. 8a) were plotted at https://onlinemathtools.com/generate-koch-snowflake. Aldus Super 3D 2.5 Macintosh OS 9.2 heritage software originally developed by Silicon Beach Software was used to construct the 3D models of Pteridinium and Ernietta shown in Figures 3, 9, 10 and 12. Illustrated specimens are in the following institutional collections: RAS PIN – Borissiak Palaeontological Institute, Russian Academy of Sciences, Moscow; SAM P – Palaeontology, South Australian Museum, Adelaide; UCLA – Earth, Planetary and Space Sciences, University of California, Los Angeles; or in private hands (Fig. 9a).
Acknowledgements
I thank Sören Jensen and his colleagues for the invitation to participate in IMECT 2019 and for hinting that a retrospective article of this kind might be an acceptable contribution to the meeting and the symposium volume. My knowledge of Ediacaran biotas has been gained through ˜40 wonderful years working with Jim Gehling. Adolf (Dolf) Seilacher generously invited Jim and I to participate in his team’s excavation of the Pteridinium bed on Aar Farm in 1993. Funding over the years has been provided by the Australian Research Grants Scheme, the U.S. National Science Foundation, the NASA Astrobiology Institute, the University of New England and UCLA. For generous discussion and advice, access to materials and vital assistance with field work I also thank Martin Glaessner, Mary Wade, Richard Jenkins, Neville Pledge, Christine Runnegar, Don Boyd, Chris Collins, Misha Fedonkin, Robert Horodyski, Gerard Germs, C. K. (Bob) Brain, Wilfried and Helmut Erni, Charles Hoffmann, Beverly Saylor, John Almond, Hans Pflug, Trevor Ford, Helen Boynton, Ben Bland, Mary Droser, Sören Jensen, Matt Saltzman, Lars Holmer, Kevin Peterson, Roger Summons and David Gold. Graham Budd and an anonymous reviewer kindly pointed out some serious problems with the submitted manuscript.