In fish, skeletal muscle, accounting for 40–60 % of body mass(Reference Valente, Moutou and Conceicao1), is the main edible part for humans. Its growth rate determines the production efficiency of fish farming and is an important economic indicator in aquaculture industry. Fish skeletal muscle growth involves both the hyperplasia (increase in amount) and the hypertrophy (enlargement of volume) of muscle fibres(Reference Valente, Moutou and Conceicao1). These processes are much sensitive to body nutritional state. Increasing evidence suggests that they can be widely influenced by both the quantity and quality of various nutrients, including protein(Reference Wei, Li and Xu2,Reference Silva, Valente and Galante3) , lipid(Reference Huang, Jiang and Abasubong4,Reference Lopes, Castro and Valente5) , carbohydrates(Reference Huang, Jiang and Abasubong4,Reference de Oliveira-Júnior, de Aguiar and Carneiro6) , amino acids(Reference Alami-Durante, Bazin and Cluzeaud7–Reference Sampath, Zhang and Liu9), amino acid derivatives(Reference White, Baumgarner and Watanabe10), nucleotides(Reference Asaduzzaman, Ikeda and Abol-Munafi11) and vitamins(Reference Zanella, Magiore and Duran12,Reference Alami-Durante, Cluzeaud and Bazin13) , etc. However, limited information is available on the effects of mineral nutrients.
Se is an essential mineral element for fish to maintain the normal physiological progress(Reference Prabhu, Schrama and Kaushik14). Its deficiency leads to a variety of physiological disorders, such as redox imbalance(Reference Bell, Cowey and Adron15), inflammation response(Reference Gao, Tang and Liang16) and impaired structural integrity(Reference Zheng, Jiang and Feng17), retarding the normal growth and development of fish tissue and organs(Reference Wang, Zhang and Wu18–Reference Zhang, Teng and Wang20). For fish skeletal muscle, a few researches in rainbow trout (Oncorhynchus mykiss) and zebrafish (Danio rerio) demonstrated that its growth rate is much sensitive to the change of body Se status and exhibits a clearly decrease once fish suffer from Se deficiency. The results of histological identification suggest that Se deficiency-caused growth retardation of fish skeletal muscle is primarily attributed to the suppressed muscle fibre hypertrophy(Reference Wang, Yin and Zhang19–Reference Wang, Zhang and Li21). However, the underlying mechanisms are still not well studied.
Protein accretion, resulting from the rate of protein synthesis exceeding that of protein degradation, is an important approach for muscle fibre hypertrophy(Reference Valente, Moutou and Conceicao1). In fish skeletal muscle, protein synthesis is primarily under the control of insulin-like growth factors-mediated protein kinase B (PKB/Akt)-target of rapamycin complex 1 (TORC1) pathway, and protein degradation is regulated by multiple pathways including calpain system, ubiquitin–proteasome and autophagy–lysosome pathways.(Reference Johnston, Bower and Macqueen22) Researches those focus on the effect of Se deficiency on the basal (fish were deprived of food for 24 h) protein synthesis and degradation and their regulatory pathways in rainbow trout skeletal muscle suggested Se deficiency accelerated ubiquitin-proteasome-mediated protein degradation, while exerted no influence on TORC1 pathway and protein synthesis(Reference Zhang, Teng and Wang20,Reference Wang, Zhang and Li21) . However, protein accretion and the relevant regulatory pathways in fish skeletal muscle always dynamically change after a meal(Reference Seiliez, Panserat and Skiba-Cassy23–Reference Xu, He and Mai25). A later postprandial dynamics research (throughout 0–24 h after a meal) in rainbow trout skeletal muscle reported that Se deficiency just suppressed the activation of TORC1 pathway while exerted no significant impact on protein degradation-related pathways, strongly supporting a new speculation that Se deficiency-caused disorder of muscle fibre hypertrophy in fish is primarily attributed to the impaired TORC1-mediated protein synthesis(Reference Wang, Zhang and Li26).
Within the nutritional dose range, Se primarily incorporates into various selenoproteins (Seleno) as selenocysteine to exert its biological activity(Reference Lu and Holmgren27). Se deficiency leads to an extensive down-regulation of stress-sensitive selenoproteins(Reference Mariotti, Ridge and Zhang28). In fish skeletal muscle, Se deficiency has been reported to decrease mRNA levels of multiple selenoprotein genes, such as glutathione peroxidase (gpx) 1, gpx4, selenou, selenow, selenop and selenok.(Reference Wang, Zhang and Wu18,Reference Wang, Yin and Zhang19,Reference Wang, Zhang and Li21) It is well known that these genes encode antioxidative selenoproteins(Reference Papp, Lu and Holmgren29,Reference Jiang, Huang and Lin30) . Down-regulation of them has been reported to be associated with decreased antioxidative capacity and increased reactive oxygen species (ROS) concentration in various tissues and cell types(Reference Huang, Li and Zhao31–Reference Tang, Li and Zhang35). In skeletal muscle, high concentration of ROS has been widely demonstrated as an inhibitor of TORC1-mediated protein synthesis(Reference Gomez-Cabrera, Arc-Chagnaud and Salvador-Pascual36). An important junction between high concentration of ROS and TORC1-mediated protein synthesis is Akt, which is activated upon its phosphorylation at Thr308 and Ser473(Reference Manning and Toker37). The oxidation of Akt by high concentration of ROS does not directly affect its kinase activity but promotes it dephosphorylation by protein phosphatase 2A(Reference Tan, Shavlakadze and Grounds38). In fish, Se deficiency-caused inhibition of Akt phosphorylation at Thr308 and Ser473 has been extensively observed in skeletal muscle of various fish species(Reference Wang, Yin and Zhang19,Reference Zhang, Teng and Wang20) , suggesting an inhibiting effect of Se deficiency on Akt activity, which might attribute to the deficiency-caused retardant of TORC1-mediated protein synthesis in fish skeletal muscle.
As mentioned above, there is a hypothesis that Se deficiency-caused high concentration of ROS suppresses TORC1-mediated protein synthesis by inhibiting Akt, thereby retarding the normal hypertrophy of muscle fibres, which leads to a suppressed growth of fish skeletal muscle. This study was conducted in zebrafish, an ideal model fish for nutrition and growth(Reference Ulloa, Iturra and Neira39), to test this hypothesis. The findings of this study could provide a mechanistic explanation for Se deficiency-caused suppression of muscle fibre hypertrophy in fish and help to better understand the nutritional necessity and regulatory mechanisms of Se in fish skeletal muscle growth.
Materials and methods
Ethical approval
The present experiment was approved by the Scientific Ethics Committee of Huazhong Agricultural University (approval number: HZAUFI-2022–0008), Wuhan, China.
Experimental diet
Six experimental diets (Table 1), among which three are Se-deficient and the other three are Se-adequate for juvenile zebrafish, were prepared referring to our previous report(Reference Wang, Yin and Zhang19). The basal Se-deficient diet (DSe) was formulated with low Se ingredients and reached a total Se level of 0·05 mg/kg. The basal Se-adequate diet (ASe) was prepared by supplementing the basal Se-deficient diets with Se-enriched yeast (Angel Yeast Co., Ltd) to reach the total Se level of 0·36–0·37 mg/kg. All the diets were pre-supplemented with 100 mg/kg DL-α-tocopherol acetate, named as VE, via a vitamin premix to meet the requirement of zebrafish(Reference Motorykin, Traber and Tanguay40). To access the potential effect of Se deficiency-induced oxidative stress on skeletal muscle growth of zebrafish, the basal Se-deficient and Se-adequate diets were further supplemented with extra DL-α-tocopherol acetate (1000 mg/kg) as antioxidant and named as DSe + VE and ASe + VE, respectively. To access the role of TORC1-mediated protein synthesis in the regulation of Se on zebrafish skeletal muscle growth, the basal Se-deficient and Se-adequate diets were supplemented with TOR activator (1000 mg/kg MHY1485) and named as DSe + MHY1485 and ASe + MHY1485, respectively. The detailed procedure for diet preparation referred to our previous report(Reference Wang, Zhang and Wu18). The obtained diet pellets (particle size: 0.36–0.50 mm) were freeze-dried and stored at –20°C until use. The proximate composition and total Se content of experimental diets were measured as previously described(Reference Wang, Zhang and Wu18) and presented in Table 1.
Table 1. Feed ingredients and proximate composition of experimental diets (g/kg diet)
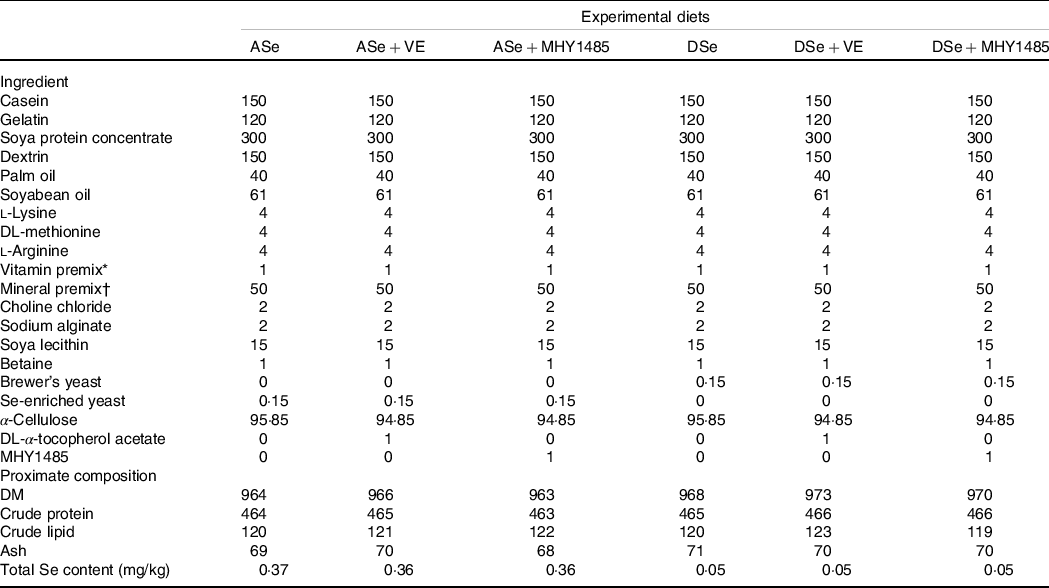
ASe, the basal selenium-adequate diet; DSe, the basal selenium-deficient diet; MHY1485, activator of the target of rapamycin; VE, DL-α-tocopherol acetate, antioxidant.
* Vitamin premix (mg/kg diet): retinol acetate, 7·30, thiamine, 15·00; lactoflavine, 15·00; nicotinic acid, 200·00; D-calcium panthothenate, 60·00; pyridoxine-HCl, 20·00; D-biotin, 1·00; folic acid, 5·00; cyanocobalamin, 0·03; l-ascorbic acid, 123·00; cholecalciferol, 4·00; DL-α-tocopherol acetate, 100·00; menadione, 5·00; inositol, 400·00; α-cellulose, 44·67.
† Mineral premix (mg/kg diet): Mg(OH)2, 5000·00; FeSO4·7H2O, 400·00; KI, 10·00; ZnSO4·7H2O, 200·00; CuSO4·5H2O, 10·00; MnSO4·H2O, 80·00; CaHPO4·2H20, 20000·00; CoCl2·6H2O, 2·00; KCl, 15000·00; NaCl, 4298·00; α-cellulose, 5000·00.
Experimental design and feeding trial
Juvenile zebrafish (30 d post-fertilisation, dpf) were obtained from the Chinese National Zebrafish Resource Center (Wuhan, China) and maintained in a zebrafish breeding system under a 14-h light/10-h dark cycle at 28°C. Zebrafish were fed the DSe for 15 d to acclimatise to the experimental diets. Then, a total of 720 sexually immature zebrafish (45 d post-fertilisation (dpf)) of similar size (mean ± sem body weight: 44·89 ± 0·08 mg, mean ± sem body length: 13·48 ± 0·02 mm, data were obtained from thirty randomly selected fish) were randomly allocated into six treatment groups with three replicates of forty fish per tank (30 litres, in a recirculating system) in each. The six experimental diets were randomly allocated in triplicate tanks (n 3). Fish were hand-fed to apparent satiation twice daily (09.00 and 16.00). During the experimental period, the dissolved oxygen and Se concentration in the water were maintained at 7·7–8·4 mg/l and < 0·3 μg/l, respectively.
Sampling procedure
After 30 d of feeding, fish were deprived of food for 24 h, and the final body weight and length were measured individually. Then, fifteen fish from each tank were randomly sampled for the analysis of the Se concentration, Gpx activity, selenoprotein gene expression, ROS concentration and the levels of oxidative status biomarkers within dorsal muscle. Another three fish were randomly sampled for histological characterisation of muscle tissue at the vent level. Subsequently, the remaining fish were refed once with the allocated diets until visible satiation. Six fish from each tank were randomly caught for the determination of protein synthesis rate and phosphorylation levels of proteins related to protein synthesis in dorsal muscle at 2 h after the meal. Prior to sampling, fish were euthanised on ice. After sampling, the samples for the histological characterisation and the measurement of ROS concentration were immediately handled, while the samples for other indicators were quickly frozen, powdered in liquid N2 and stored at −80°C until analysis. The histological characterisation was performed on three individuals from each tank, while the other indicators were measured on a pool of three fish per tank.
Determination of selenium status in zebrafish skeletal muscle
The change of Se status in skeletal muscle of zebrafish was analysed by detecting total Se concentration, Gpx activity and mRNA levels of selenoprotein genes.
The total Se concentration in zebrafish skeletal muscle was measured according to our previous report(Reference Wang, Yin and Zhang19).
The Gpx activity in zebrafish skeletal muscle was determined using a commercial detection kit (A005–1, Nanjing Jiancheng Bioengineering Institute) by measuring the GSH substrate. The result is expressed as nmol GSH per min per mg protein.
The mRNA levels of thirty-seven selenoprotein genes in zebrafish skeletal muscle were measured using quantitative real-time PCR (qPCR) method. The detailed description for the extraction of total RNA, synthesis of complementary DNA, qPCR procedure and specific primers for zebrafish selenoprotein genes referred to our previous report(Reference Wang, Yin and Zhang19). The relative quantification of the target gene was performed using the mathematical model described by Pfaffl(Reference Pfaffl41) and normalised to eukaryotic translation elongation factor 1α 1, like 1 (eef1a1l1).
Histological characterisation
Whole-fish transverse sections were cut at the vent level. The frozen slices were made and stained with fluorescein isothiocyanate-conjugated wheat germ agglutinin (FITC-WGA, GeneTex) as our previous report(Reference Wang, Yin and Zhang19). Images of transverse sections of muscle tissue and muscle fibres were obtained by scanning the slices using a Pannoramic 250 Flash digital microscope (3DHISTECH). In fish, white muscle is the predominant component, accounting for > 70 % of mass, of skeletal muscle(Reference de Paula, de Almeida and Carani42). Thus, the histological characterisation of zebrafish skeletal muscle in this study mainly focused on white muscle. The total cross-sectional area of white muscle and the individual equivalent mean diameter of white muscle fibres from the whole-fish transverse section were measured as previously described(Reference Wang, Zhang and Li21).
Determination of reactive oxygen species concentrations and levels of oxidative status biomarkers
The ROS concentrations in zebrafish skeletal muscle were determined with various fluorescent probes. Prior to determination, fresh muscle tissue were homogenised 1:10 (w/v) in a buffer consisting of 25 mmol/l TRIS hydrochloride (pH 7·4), 1 mmol/l EDTA and 1 mmol/l EGTA(Reference Mason, Della Gatta and Snow43). Then, the concentration of non-specific ROS was measured as previously described(Reference Fu and Ji44) with modification. Briefly, 10 μl of homogenate was incubated with 190 μl 2’,7’-dichlorodihydrofluorescein diacetate (5 μmol/l final concentration) at 37°C for 30 min in the dark. The fluorescence intensity was detected with an excitation wavelength of 488 nm and an emission wavelength of 525 nm. The concentration of hydrogen peroxide (H2O2) was measured as previously described(Reference Kim, Choi and Park45) with modification. Briefly, 10 μl of homogenate was incubated with 140 μl mixture of Amplex red (10 mmol/l final concentration) and horseradish peroxidase (0·1 U/ml final concentration) at 37°C for 30 min in the dark. The fluorescence intensity was detected with an excitation wavelength of 530 nm and an emission wavelength of 590 nm. The concentration of superoxide anion (•O2 −) was measured by incubating the homogenate with dihydroethidium at 37°C for 30 min in the dark, and the fluorescence intensity was detected with an excitation wavelength of 535 nm and an emission wavelength of 610 nm. In this study, the fluorescence intensity was detected using a SpectraMax i3x Multi-Mode Microplate Reader (Molecular Devices). According to these three methods, the rates of changes in sample fluorescence were all linear for at least within 60 min over a range of protein concentrations (from 0- to 10 000-fold dilution of the homogenate).
The levels of oxidative status biomarkers including malondialdehyde and protein carbonyl in zebrafish skeletal muscle were determined by the corresponding detection kits (A003–1 and A087–1) purchased from Nanjing Jiancheng Bioengineering Institute.
Determination of phosphorylation levels of proteins related to protein synthesis
The phosphorylation levels of key proteins in Akt-TORC1 pathway, which is involved in protein synthesis, were measured by Western blotting assay. The detailed procedure referred to our previous report(Reference Wang, Zhang and Li21). The specific primary antibodies against Akt, phospho (p) -Akt (Thr308), p-Akt (Ser473), S6 ribosomal protein (S6), p-S6 (Ser235/236), eukaryotic translation initiation factor 4E binding protein (4E-BP1), p-4E-BP1 (Thr37/46), eukaryotic translation elongation factor 2 (eEF2), p-eEF2 (Thr56), the target of rapamycin (TOR), p-TOR (Ser2448), 70 kDa ribosomal protein S6 kinase (S6K1), p-S6K1 (Thr389) referred to previous researches in zebrafish(Reference Wang, Yin and Zhang19,Reference Tian, Wang and Wang46) . The primary antibody against α-Tubulin (Cat. no. 2125) was purchased from Cell Signaling Technology Inc., and the antigenic amino acid sequence was monitored in the NCBI database (https://blast.ncbi.nlm.nih.gov/Blast.cgi) to check for a good conservation of the target protein.
Determination of protein synthesis rate
The protein synthesis rate in zebrafish skeletal muscle was measured according to the principles of the surface sensing of translation method as described previously(Reference Wang, Yin and Zhang19). Briefly, after the last meal, three fish from each tank were randomly selected and immediately exposed to 0·04 mmol/l puromycin (Sigma-Aldrich) for 2 h. Then, the dorsal muscle was sampled to quantify the puromycin-labelled peptides, which can be measured by Western blotting assay using a specific primary antibody against puromycin (clone 12D10, Cat. no. MABE343-AF647, Millipore).
Statistical analysis
All data are presented as mean ± sem and statistically analysed using SPSS 26.0 (SPSS Inc.). Shapiro–Wilk test and Levene’s test were performed to test the normal distribution and homogeneity of the variances, respectively. Data with non-normal distribution were subjected to logarithmic (Log10) or square root (SQRT) transformations. Then, data were subjected to a two-way (dietary Se × dietary VE or MHY1485) ANOVA followed by a Bonferroni–Dunn multiple comparison. The level of significance was set at P < 0·05.
Results
Effects of dietary selenium, VE and MHY1485 on body weight and body length of zebrafish
Throughout the feeding trial, no deaths were observed. After 30 d of feeding, the final body length of zebrafish showed no significant difference among groups, while the final body weight was significantly affected by dietary treatments (Fig. 1). Dietary Se deficiency led to a significant decrease of final body weight by 9·58 % as shown in the difference between ASe and DSe groups (P < 0·05). Dietary supplementation of VE and MHY1485 exerted no significant influences on the final body weight of zebrafish fed Se-adequate diets, whereas significantly increased (P < 0·05) the final body weight of zebrafish fed Se-deficient diets to the comparable levels in zebrafish fed the Se-adequate diets.
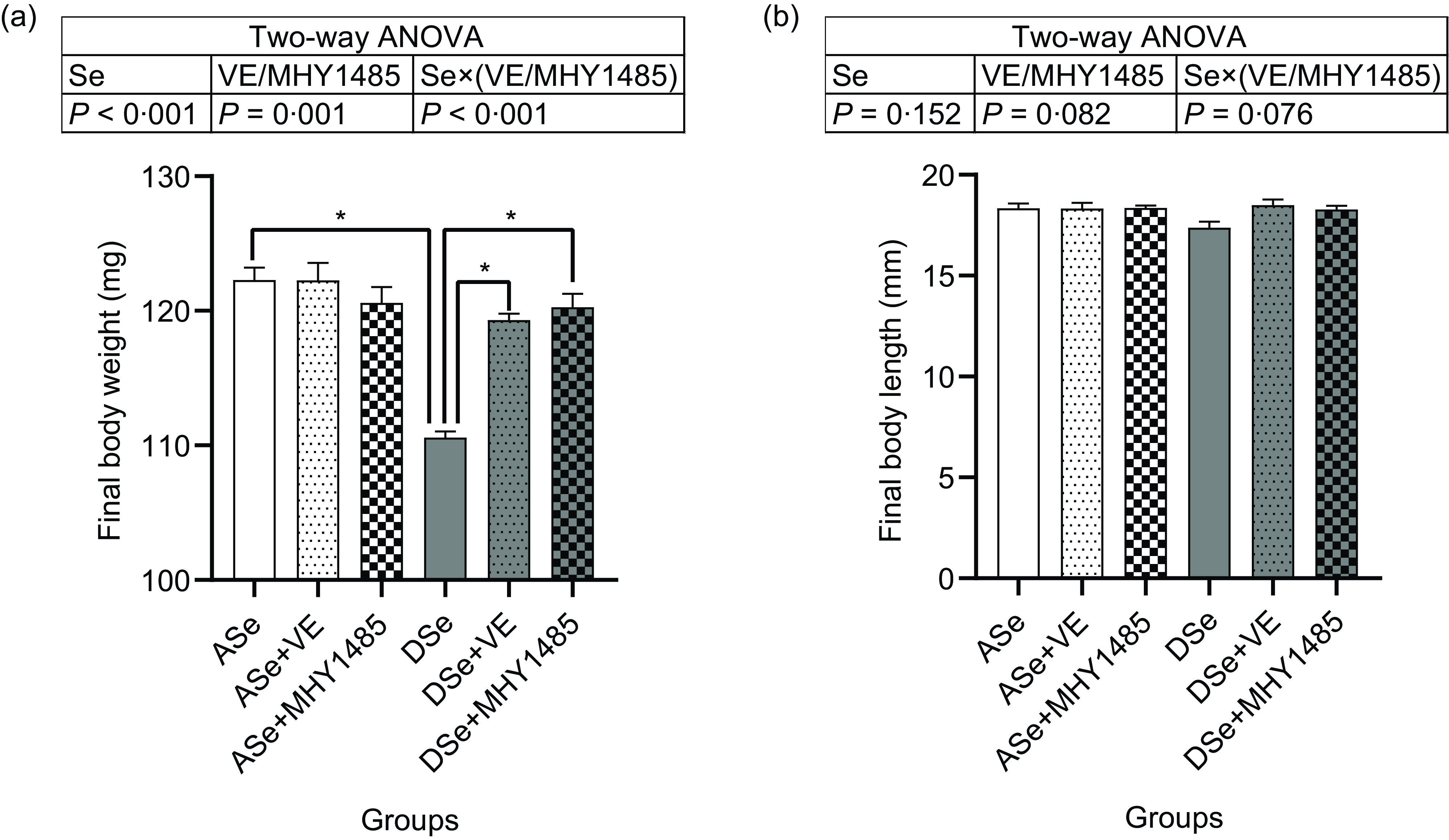
Fig. 1. Effects of 30 d of dietary treatments on (a) body weight and (b) body length of juvenile zebrafish. Values are means ± sem, n 3. *Significantly different (P < 0·05, two-way (dietary Se × dietary VE or MHY1485) ANOVA followed by Bonferroni–Dunn multiple comparison). ASe, the basal Se-adequate diet; DSe, the basal Se-deficient diet; VE, DL-α-tocopherol acetate, antioxidant; MHY1485, activator of the target of rapamycin.
Effects of dietary selenium, VE and MHY1485 on selenium status in skeletal muscle of zebrafish
The change of Se status in skeletal muscle of zebrafish fed different experimental diets was comprehensively evaluated by analysing the total Se concentration and the levels of Se status biomarkers, Gpx activity and transcript abundances of thirty-seven selenoprotein genes, within muscle tissue. The results of two-way ANOVA showed that the total Se concentrations, Gpx activities and mRNA levels of eleven selenoprotein genes (dio1, gpx1a, gpx3, gpx4a, selenoj, selenok, selenol, selenop1a, selenot2, selenou1a and selenow1) significantly decreased (P < 0·05) in zebrafish fed Se-deficient diets (DSe, DSe + VE and DSe + MHY1485) compared with those fed Se-adequate diets (ASe, ASe + VE and ASe + MHY1485, Fig. 2). However, most of these biomarkers exhibited no significant differences among fish fed Se-adequate diets (ASe, ASe + VE and ASe + MHY1485) or among zebrafish fed Se-deficient diets (DSe, DSe + VE and DSe + MHY1485, Fig. 2). These results suggest Se status in zebrafish skeletal muscle was only affected by dietary Se level but not by dietary VE or MHY1485 supplementation.
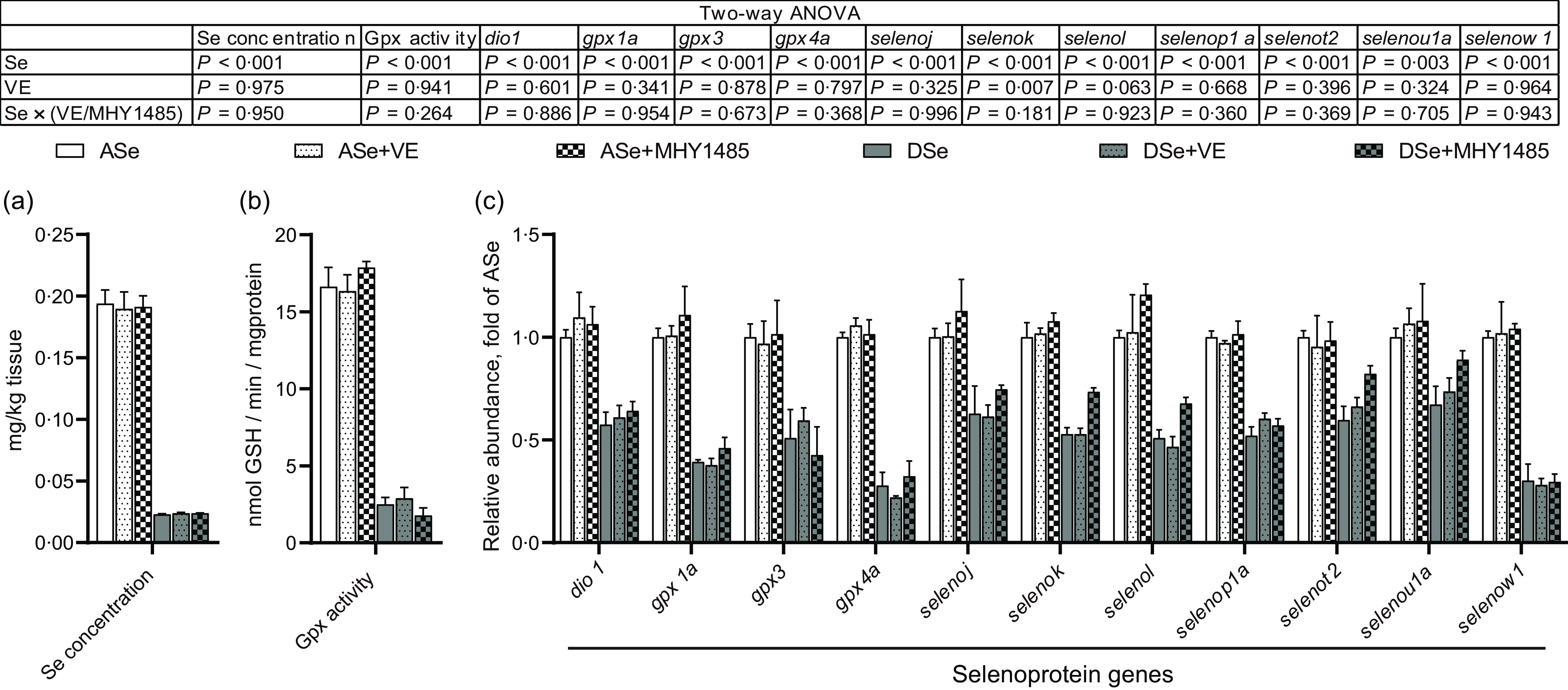
Fig. 2. Effects of 30 d of dietary treatments on Se status in skeletal muscle of juvenile zebrafish. (a) Total Se concentration. (b) Gpx activity. (c) Relative mRNA levels of selenoprotein genes. Values are means ± sem, n 3. *Significantly different (P < 0·05, two-way (dietary Se × dietary VE or MHY1485) ANOVA followed by Bonferroni–Dunn multiple comparison). ASe, the basal Se-adequate diet; DSe, the basal Se-deficient diet; VE, DL-α-tocopherol acetate, antioxidant; MHY1485, activator of the target of rapamycin; Gpx, glutathione peroxidase; dio, iodothyronine deiodinase; seleno, selenoprotein.
Effects of selenium status on reactive oxygen species concentration, Akt-TORC1 pathway, protein synthesis rate and histological characterisation in skeletal muscle of zebrafish
The effects of Se deficiency in zebrafish skeletal muscle were accessed by analysing the changes in zebrafish fed the DSe compared with those fed the ASe.
Results of ROS fluorescent probe assays showed that Se deficiency significantly increased intramuscular concentrations of non-specific ROS, •O2 − and H2O2 by 1·16, 15·49 and 23·60 times, respectively (P < 0·05, Fig. 3a). Accordingly, Se deficiency significantly elevated the levels of intramuscular protein carbonyl and malondialdehyde, two oxidative biomarkers, by 5·42 and 5·03 times, respectively (P < 0·05, Fig. 3b).
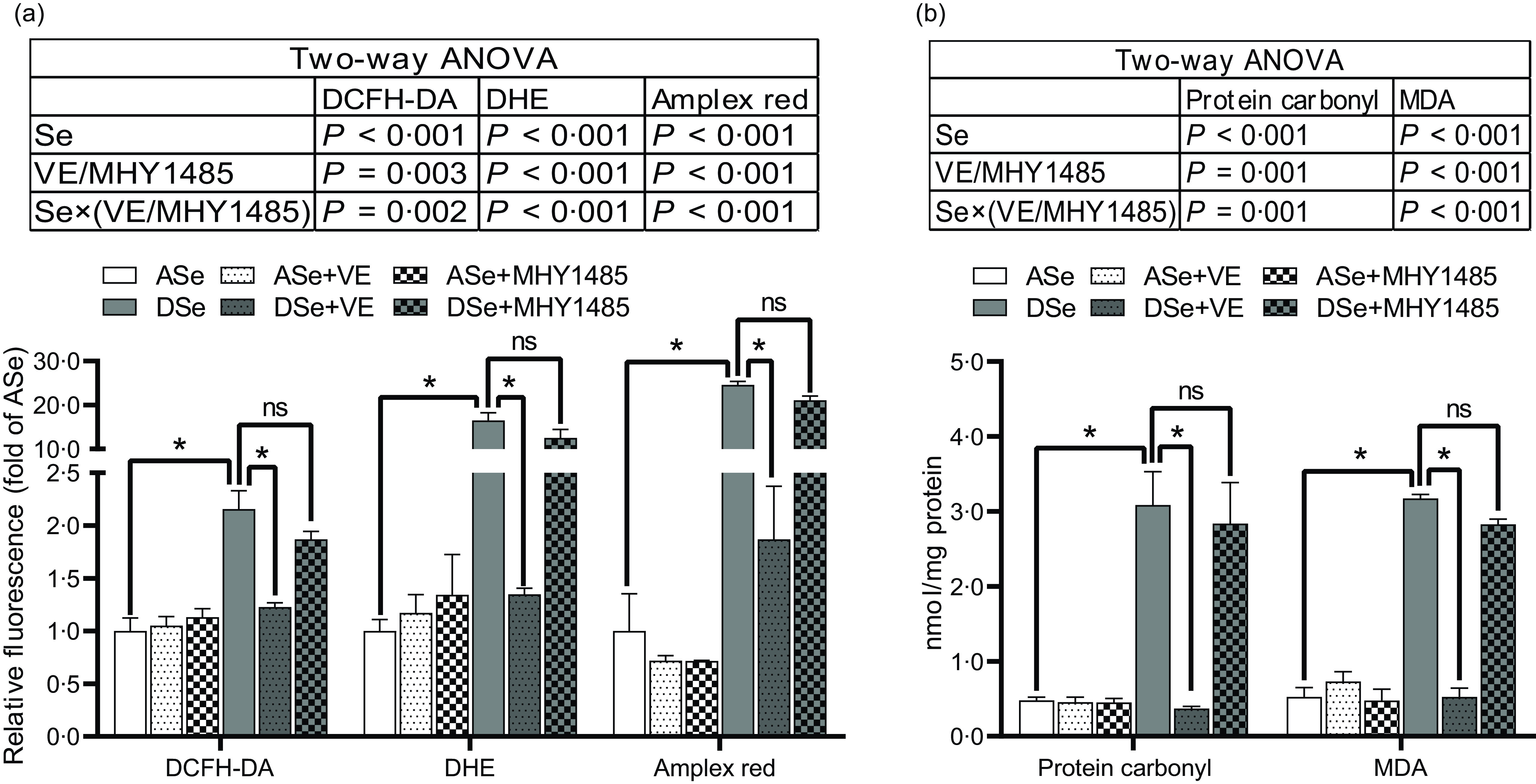
Fig. 3. Effects of 30 d of dietary treatments on the levels of (a) ROS and (b) oxidative status biomarkers in skeletal muscle of juvenile zebrafish. (a) Three different ROS fluorescent probes (DCFH-DA, DHE and Amplex red) were applied to detect non-specific ROS, superoxide anion and hydrogen peroxide, respectively. (b) The levels of protein carbonyl and malondialdehyde were detected to evaluate oxidative status. Values are means ± sem, n 3. *Significantly different, ns represents not significantly different (P < 0·05, two-way (dietary Se × dietary VE or MHY1485) ANOVA followed by Bonferroni-Dunn multiple comparison). ASe, the basal Se-adequate diet; DSe, the basal Se-deficient diet; VE, DL-α-tocopherol acetate, antioxidant; MHY1485, activator of the target of rapamycin; ROS, reactive oxygen species; DCFH-DA, 2’,7’-dichlorodihydrofluorescein diacetate; DHE, dihydroethidium; MDA, malondialdehyde.
Se deficiency significantly decreased the phosphorylation levels of Akt on Thr308 and Ser473 residues in zebrafish skeletal muscle by 69·14 % and 50·05 %, respectively (P < 0·05, Fig. 4). Se deficiency also declined the phosphorylation levels of key proteins in TORC1 pathway including TOR (Ser2448), S6K1 (Thr389), S6 (Ser235/236) and 4E-BP1 (Thr37/46) by 69·14 %, 50·05 %, 52·43 %, 57·58 %, 66·33 % and 70·79 %, respectively, and increased the phosphorylation level of eEF2 (Thr56) by 1·74 times (P < 0·05), suggesting an inhibitory effect of Se deficiency on TORC1 pathway in zebrafish skeletal muscle (Fig. 4). Be consistent with the response of Akt-TORC1 pathway to Se deficiency, protein synthesis rate in skeletal muscle of zebrafish fed the DSe decreased to 67·46 % of that in zebrafish fed the ASe (P < 0·05, Fig. 5).
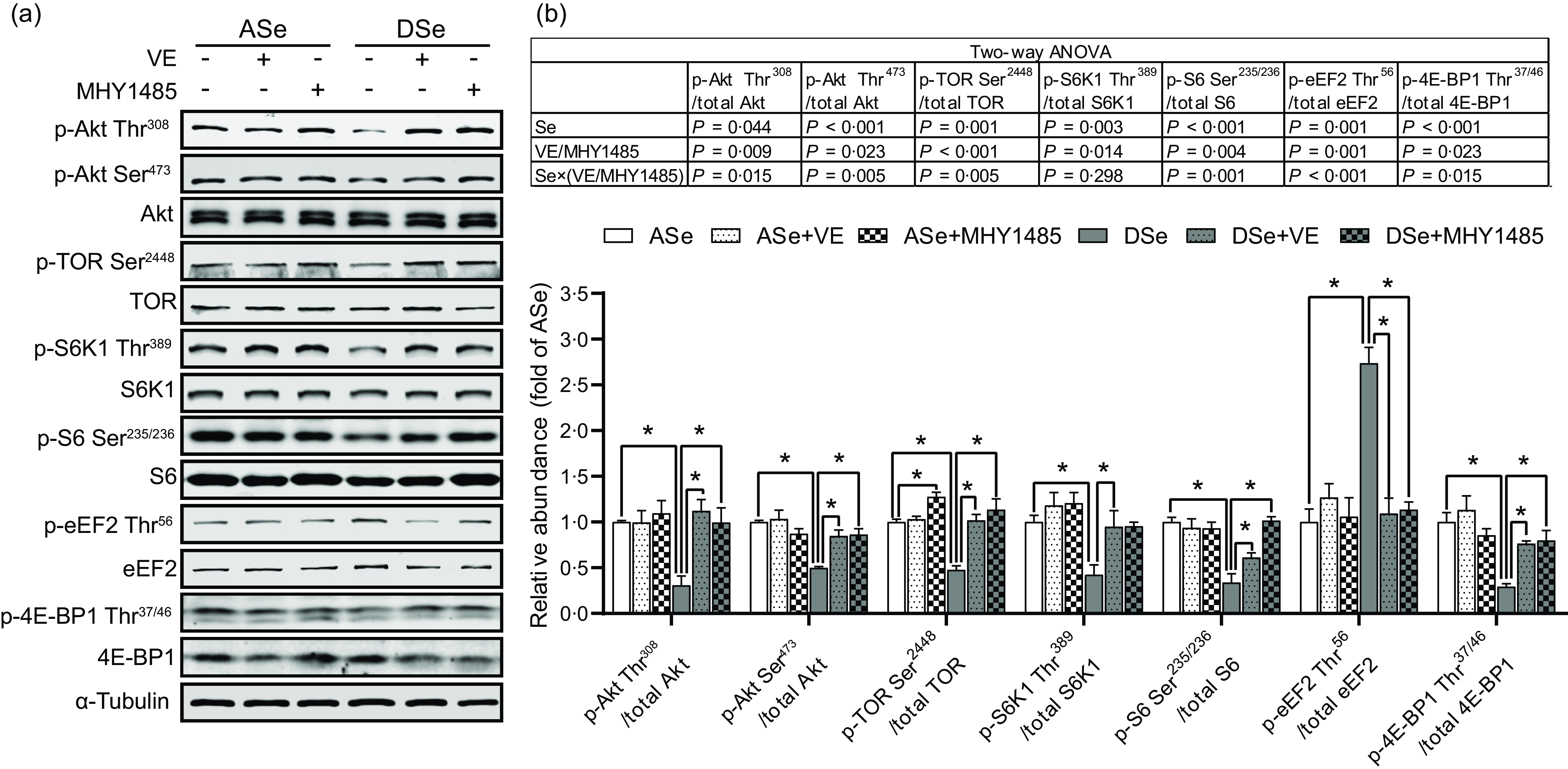
Fig. 4. Effects of 30 d of dietary treatments on Akt-TORC1 pathway in skeletal muscle of juvenile zebrafish. Data were obtained 2 h after a meal by (a) Western blotting assay, and (b) the relative phosphorylation levels of proteins in Akt-TORC1 pathway were calculated. Values are means ± sem, n 3. *Significantly different (P < 0·05, two-way (dietary Se × dietary VE or MHY1485) ANOVA followed by Bonferroni–Dunn multiple comparison). ASe, the basal Se-adequate diet; DSe, the basal Se-deficient diet; VE, DL-α-tocopherol acetate, antioxidant; MHY1485, activator of the target of rapamycin; Akt, protein kinase B; TOR, the target of rapamycin; TORC1, the target of rapamycin complex 1; S6K1, 70 kDa ribosomal protein S6 kinase; S6, ribosomal protein S6; eEF2, eukaryotic translation elongation factor 2; 4E-BP1, eukaryotic translation initiation factor 4E binding protein.
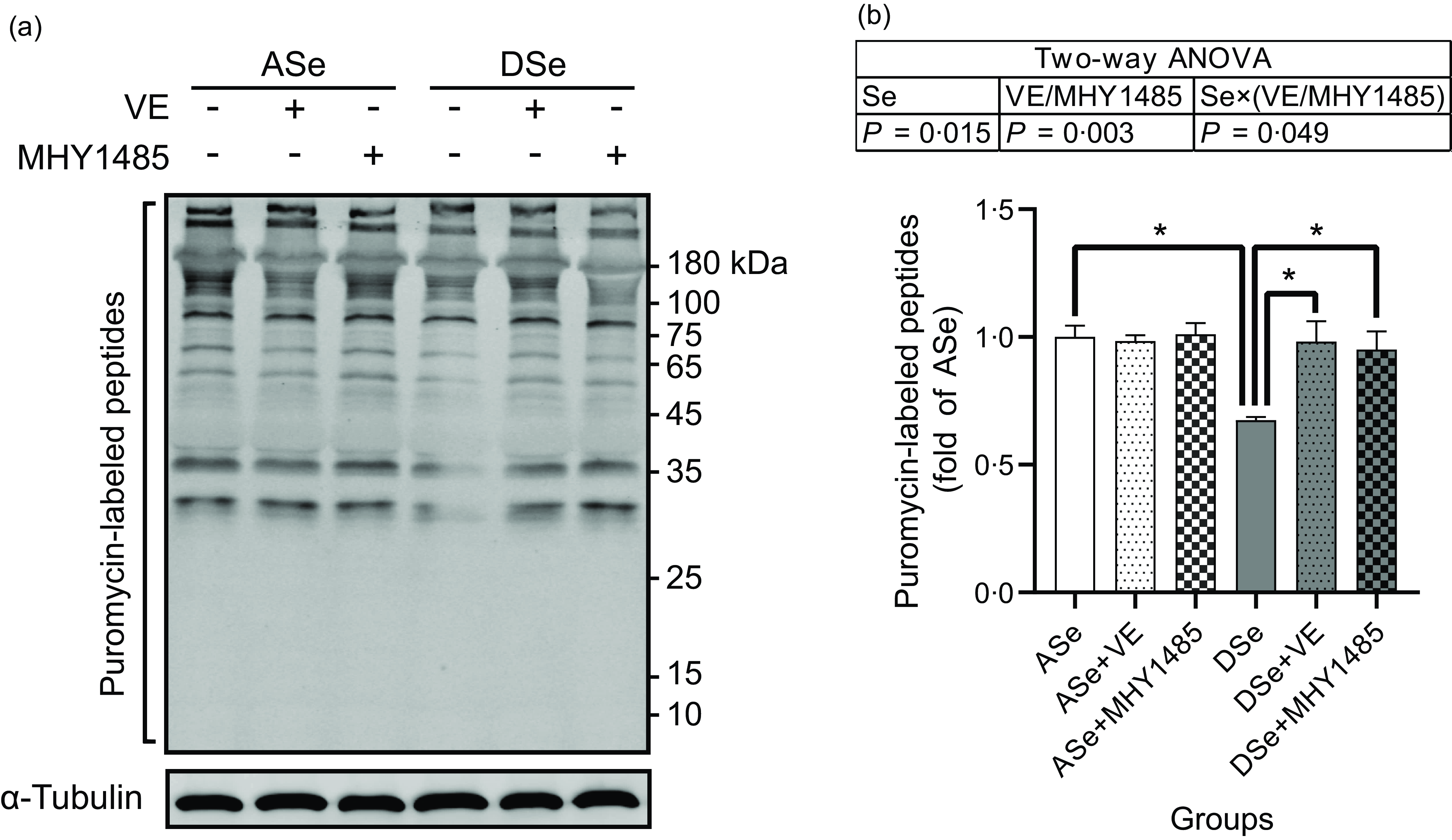
Fig. 5. Effects of 30 d of dietary treatments on protein synthesis in skeletal muscle of juvenile zebrafish. Muscle protein synthesis rate was detected 2 h after a meal using the surface sensing of translation method and quantified by measuring the incorporation of exogenous puromycin into nascent peptides. Puromycin-labelled peptides were detected by (a) Western blotting assay using an antibody against puromycin, and (b) the results are normalised to α-Tubulin. Values are means ± sems, n 3. *Significantly different (P < 0·05, two-way (dietary Se × dietary VE or MHY1485) ANOVA followed by Bonferroni–Dunn multiple comparison). ASe, the basal Se-adequate diet; DSe, the basal Se-deficient diet; VE, DL-α-tocopherol acetate, antioxidant. MHY1485, activator of the target of rapamycin.
Histological characterisation of skeletal muscle cross section at the vent level (Fig. 6) showed that Se deficiency significantly decreased the total cross-sectional area of white muscle and the individual equivalent mean diameter of white muscle fibres (P < 0·05), suggesting an inhibitory effect of Se deficiency on hypertrophic growth of zebrafish skeletal muscle.
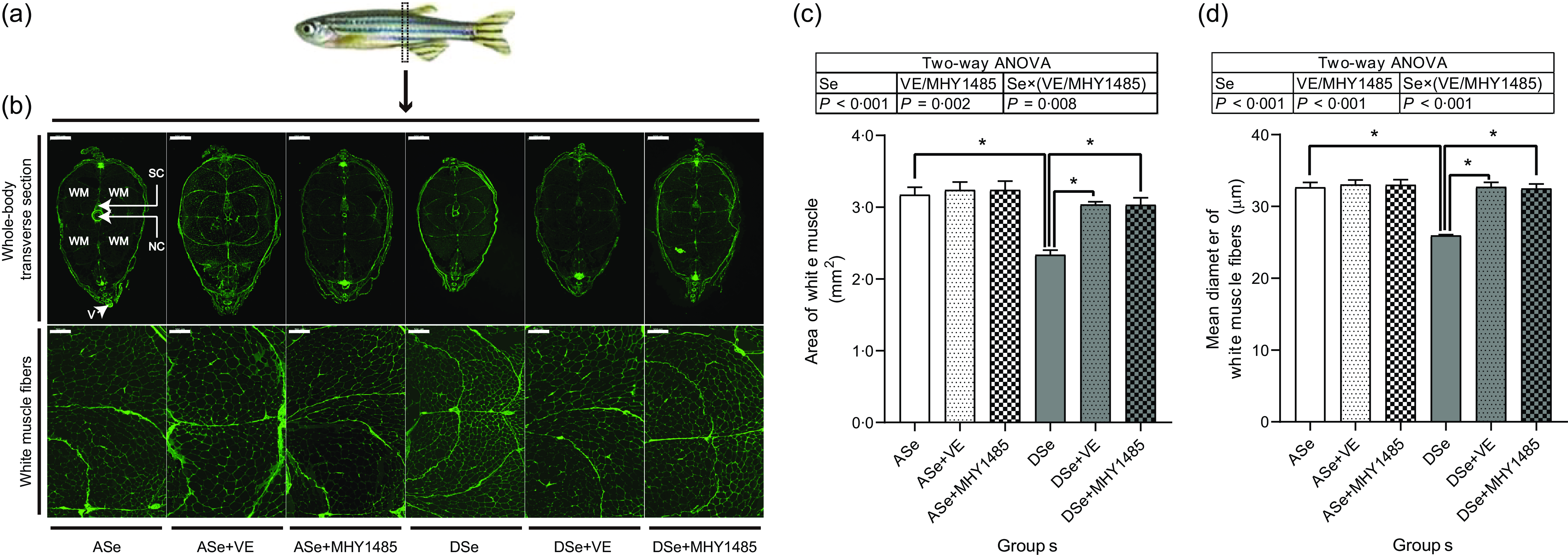
Fig. 6. Effects of 30 d of dietary treatments on histological characteristics of skeletal muscle in juvenile zebrafish. (a) Zebrafish whole-body transverse sections were obtained at the vent level and stained with wheat germ agglutinin (green, for plasma membrane). (b) Representative images of whole-body transverse sections, and the corresponding zoom-in images for white muscle fibres. The area of white muscle within the whole-body transverse section is presented in the image obtained from zebrafish fed the ASe. WM, white muscle; SC, spinal cord; NC, notochord; V, vent. Scale bar for whole-body transverse section, 500 μm; scale bar for white muscle fibre, 100 μm. Images were subjected to a morphological survey to calculate (c) the total cross-sectional area of white muscle and (d) the mean diameter of white muscle fibres. Values are means ± sem, n 3. *Significantly different (P < 0·05, two-way (dietary Se× dietary VE or MHY1485) ANOVA followed by Bonferroni–Dunn multiple comparison). ASe, the basal Se-adequate diet; DSe, the basal Se-deficient diet; VE, DL-α-tocopherol acetate, antioxidant. MHY1485, activator of the target of rapamycin.
Effects of dietary MHY1485 on TORC1 pathway, protein synthesis rate and histological characterisation in skeletal muscle of zebrafish under different selenium status
A two-way (dietary Se × dietary MHY1485) treatment was designed to access the regulatory effect of TORC1-mediated protein synthesis in Se-deficiency-induced suppression of zebrafish skeletal muscle growth.
When zebrafish were subjected to Se-adequate diets, dietary supplementation with 1000 mg/kg MHY1485 significantly elevated the phosphorylation level of TOR (Ser2448) by 27·57 % (P < 0·05, Fig. 4) and exerted no significant influences on the phosphorylation levels of downstream proteins of TOR including S6K1 (Thr389), S6 (Ser235/236), eEF2 (Thr56) and 4E-BP1 (Thr37/46) in muscle (Fig. 4), and muscle protein synthesis rate (Fig. 5) and muscle hypertrophic growth (Fig. 6).
When zebrafish were subjected to Se-deficient diets, dietary supplementation with 1000 mg/kg MHY1485 significantly elevated the phosphorylation levels of TOR (Ser2448) S6K1 (Thr389), S6 (Ser235/236) and 4E-BP1 (Thr37/46) and decreased the phosphorylation level of eEF2 (Thr56) to the similar level of each protein in zebrafish fed the ASe (P < 0·05, Fig. 4). Moreover, supplementing 1000 mg/kg MHY1485 into Se-deficient diet significantly increased muscle protein synthesis rate (Fig. 5) and improved muscle hypertrophic growth to reach the comparable levels in zebrafish fed the ASe (P < 0·05, Fig. 6).
Effects of dietary VE on reactive oxygen species concentration, TORC1 pathway, protein synthesis rate and histological characterisation in skeletal muscle of zebrafish under different selenium status
A two-way (dietary Se × dietary VE) treatment was designed to access the effects of Se-deficiency-induced high concentration of ROS on intramuscular TORC1 pathway, muscle protein synthesis and muscle hypertrophic growth.
When zebrafish were subjected to Se-adequate diets, intramuscular ROS concentrations were at relatively low levels and were not significantly influenced by dietary supplementation with 1000 mg/kg VE (Fig. 3). Accordingly, intramuscular phosphorylation levels of Akt (Thr308), Akt (Ser473), TOR (Ser2448), S6K1 (Thr389), S6 (Ser235/236), eEF2 (Thr56) and 4E-BP1 (Thr37/46), muscle protein synthesis rate and muscle hypertrophic growth exhibited no significant differences between zebrafish fed ASe + VE and ASe (Figs. 4–6).
When zebrafish were subjected to Se-deficient diets, intramuscular ROS concentrations were significantly affected by dietary supplementation of 1000 mg/kg VE. Compared with those in zebrafish fed the DSe, intramuscular concentration of non-specific ROS, •O2 − and H2O2 in zebrafish fed the DSe + VE significantly decreased (P < 0·05) and reached the similar levels observed in zebrafish fed the ASe (Fig. 3). Dietary supplementation with 1000 mg/kg VE significantly elevated the phosphorylation levels of TOR (Ser2448), S6K1 (Thr389), S6 (Ser235/236) and 4E-BP1 (Thr37/46) and decreased the phosphorylation levels of eEF2 (Thr56) to the similar level of each protein in zebrafish fed the ASe (P < 0·05, Fig. 4). Furthermore, muscle protein synthesis rate and muscle hypertrophic growth in zebrafish fed the DSe + VE were also significantly improved compared with those in zebrafish fed the DSe and reached the comparable levels in zebrafish fed the ASe (P < 0·05, Figs. 5 and 6).
Discussion
In this study, the Se-adequate level (0·36–0·37 mg/kg) and Se-deficient level (0·05 mg/kg) in experimental diets were designed according to our previous observation in juvenile zebrafish that the minimum dietary Se requirement (based on Se-enriched yeast) is 0·34 mg/kg, and less than 0·10 mg/kg dietary Se is obviously deficient for skeletal muscle(Reference Wang, Yin and Zhang19). As expected, zebrafish fed the Se-deficient diets (DSe, DSe + VE and DSe + MHY1485) exhibited clearly Se-deficient status in skeletal muscle, as evidenced by the significant decrease of total Se concentration and Se status biomarkers (Gpx activity, and mRNA of 11 selenoprotein genes: dio1, gpx1a, gpx3, gpx4a, selenoj, selenok, selenol, selenop1a, selenot2, selenou1a and selenow1) compared with those in zebrafish fed the Se-adequate diets (ASe, ASe + VE and ASe + MHY1485). Furthermore, it is worth noting that the total Se concentration and Se status biomarkers exhibited no significant differences not only among zebrafish fed the Se-adequate diets (ASe, ASe + VE and ASe + MHY1485) but also among zebrafish fed the Se-deficient diets (DSe, DSe + VE and DSe + MHY1485). These phenomena indicate that Se status in zebrafish skeletal muscle is only correlated with dietary Se level and not affected by dietary VE and MHY1485.
Histological characteristics of zebrafish skeletal muscle at the vent level showed that the total area of white muscle and muscle fibre diameter in zebrafish fed the DSe were significantly lower than those in zebrafish fed the ASe, suggesting a suppressive effect of Se deficiency on hypertrophic growth of zebrafish skeletal muscle. This phenomenon is completely consistent with previous observations both in zebrafish(Reference Wang, Yin and Zhang19) and rainbow trout(Reference Zhang, Teng and Wang20,Reference Wang, Zhang and Li21) .
It is reported that Se deficiency-caused suppressed hypertrophic growth of fish skeletal muscle is associated with the impaired TORC1 pathway-mediated protein synthesis(Reference Wang, Zhang and Li21,Reference Wang, Zhang and Li26) . In Se-deficient zebrafish, the suppressed hypertrophic growth of skeletal muscle has also been observed accompanied by the hypoactivated TORC1 pathway and impaired protein synthesis(Reference Wang, Yin and Zhang19). This phenomenon is consistent with our observation that the DSe significantly decreased intramuscular protein synthesis rate, inhibited the phosphorylation of TOR (Ser2448), S6K1 (Thr389), S6 (Ser235/236) and 4E-BP1 (Thr37/46), and promoted the phosphorylation of eEF2 (Thr56) when compared with the ASe. However, until now, it is still unclear whether Se deficiency-caused suppressed hypertrophic growth of fish skeletal muscle is directly caused by the impaired TORC1 pathway-mediated protein synthesis. In this study, we used a TOR activator (MHY1485) to activate TORC1 pathway in skeletal muscle of zebrafish fed Se-deficient diet and observed the activation of TORC1 pathway almost completely alleviated the inhibiting effects of Se deficiency on muscle protein synthesis and hypertrophic growth of skeletal muscle, as shown in zebrafish fed ASe, DSe and DSe + MHY1485. These phenomena strongly support that the impaired TORC1 pathway-mediated protein synthesis is a determinant for the suppressed hypertrophic growth of zebrafish skeletal muscle.
In eukaryotic cells, the activation of TORC1 pathway is controlled by multiple upstream protein kinases(Reference Huang and Fingar47), among which Akt is much sensitive to the change of Se status(Reference Zhang, Xia and Xia33,Reference Yang, Hamid and Cai48,Reference Wang, Lian and He49) . In this study, the phosphorylation levels of Akt at both Thr308 and Ser473 residues significantly decreased in skeletal muscle of zebrafish fed the DSe compared with the ASe, which is consistent with previous observations in fish(Reference Wang, Yin and Zhang19,Reference Zhang, Teng and Wang20) , mammals(Reference Huang, Wu and Zeng50) and poultry(Reference Gao, Nie and Wang51). Given that Akt is activated upon its phosphorylation at Thr308 and Ser473 residues(Reference Manning and Toker37), the observed suppression of Akt phosphorylation at Thr308 and Ser473 in zebrafish skeletal muscle indicates an inhibiting effect of Se deficiency on Akt activity, which might contribute to the impaired TORC1 pathway-mediated protein synthesis in zebrafish skeletal muscle.
Se deficiency has been reported to downregulate the expression of multiple selenoprotein genes those code antioxidant selenoproteins, including Gpx1, Gpx4, Selenok, Selenop, Selenou and Selenow in fish skeletal muscle(Reference Wang, Zhang and Wu18,Reference Wang, Yin and Zhang19,Reference Wang, Zhang and Li21) . A similar phenomenon has also been observed in this study that the mRNA level of gpx1a, gpx4a, selenok, selenop1a, selenou1a and selenow1 significantly decreased in skeletal muscle of zebrafish fed the DSe compared with those fed the ASe. Downregulated expression of these selenoprotein genes has been reported to be associated with decreased antioxidative capacity and increased ROS concentrations(Reference Huang, Li and Zhao31–Reference Zhang, Xia and Xia33,Reference Zhao, Sun and Huang52) . Be consistent with these reports, compared with the ASe, the DSe leads to a significant increase of ROS concentrations, thereby causing oxidative stress in zebrafish skeletal muscle evidenced by the elevated levels of protein carbonyl and malondialdehyde, two biomarkers for oxidative status(Reference Kadiiska, Gladen and Baird53).
In skeletal muscle, stresses- and diseases-induced high concentrations of ROS have been widely demonstrated to suppress TORC1 pathway-mediated protein synthesis by regulating the activity of Akt(Reference Gomez-Cabrera, Arc-Chagnaud and Salvador-Pascual36). However, it is still unclear whether there exists a same regulatory effect of Se deficiency-induced high concentrations of ROS. Given that Se deficiency did increase ROS concentrations, inhibit Akt activity and impair TORC1 pathway-mediated protein synthesis in zebrafish skeletal muscle. An antioxidant (VE) was further supplemented to the diets to access the direct action of high concentrations of ROS in Se deficiency-caused suppression of Akt activity and TORC1 pathway-mediated protein synthesis. As shown in zebrafish fed the DSe + VE compared with those fed the DSe, the decrease in ROS concentrations in zebrafish skeletal muscle by VE alleviated the inhibiting effects of Se deficiency on Akt phosphorylation at Thr308 and Ser473. Accordingly, Se deficiency-caused suppression of TORC1 pathway, protein synthesis and hypertrophic growth of skeletal muscle have also been alleviated. These evidences strongly support that Se deficiency-caused high concentration of ROS exerts a direct inhibiting effect on TORC1 pathway-mediated protein synthesis by inhibiting Akt, thereby resulting into an impaired hypertrophic growth of zebrafish skeletal muscle.
It is noteworthy that VE, the antioxidant used in this study, is also an essential nutrient for fish. The elevated level of VE in zebrafish diets might exert potential nutritional benefits for zebrafish skeletal muscle growth, which in turn interfere the evaluation in this study. However, in this study, all experimental diets have been presupplemented with 100 mg/kg VE via a vitamin premix to meet the requirement of zebrafish(Reference Motorykin, Traber and Tanguay40). Therefore, the extra-supplemented VE (1000 mg/kg) exerted no obvious nutritional benefits for zebrafish skeletal muscle when dietary Se is adequate, as shown in zebrafish fed the ASe and the ASe + VE. It primarily acted as an antioxidant to remove the excess ROS when dietary Se is deficient, as shown in zebrafish fed the DSe and the DSe + VE.
In summary, this study demonstrates that Se deficiency-induced high concentration of ROS impairs TORC1 pathway-mediated protein synthesis by inhibiting Akt activity, thereby leading to a suppression on muscle fibre hypertrophy and retarding skeletal muscle growth in zebrafish (as shown in Fig. 7). These results provide a mechanistic explanation for Se deficiency-caused suppression in hypertrophic growth of fish skeletal muscle, which contributes to the growing recognition of the nutritional role and mechanisms of Se in fish skeletal muscle biology.
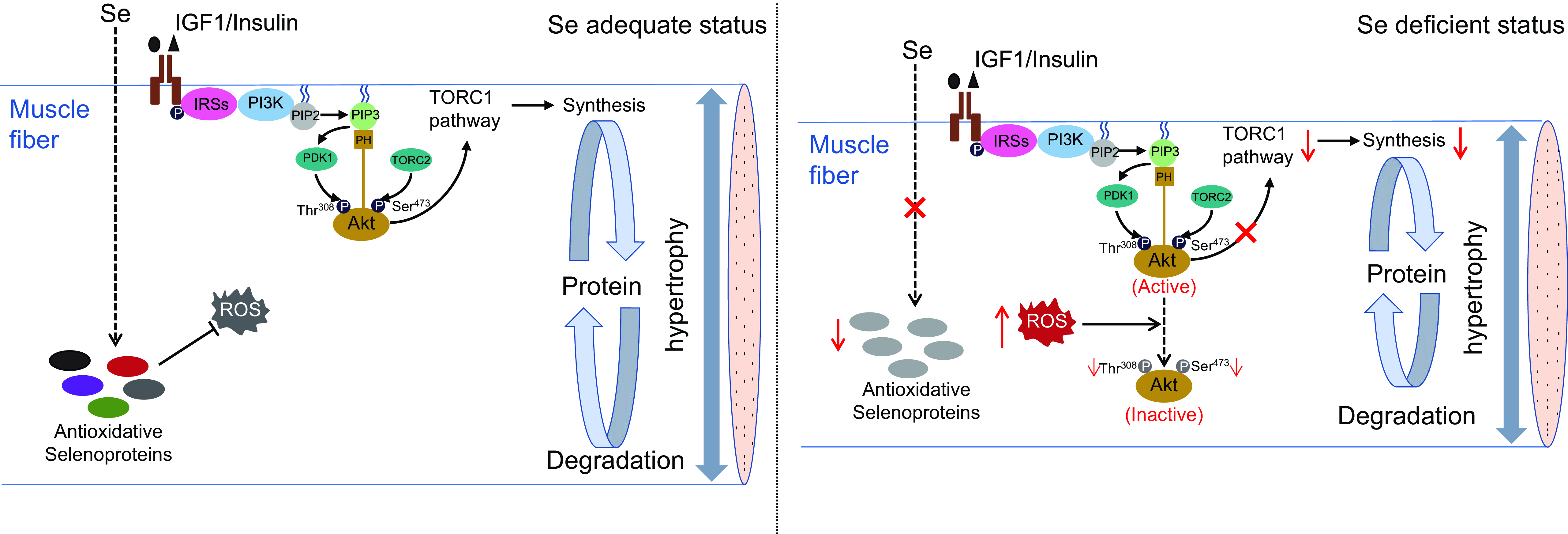
Fig. 7. A schematic diagram for the regulatory mechanism of Se in skeletal muscle fibre hypertrophy in zebrafish. (a) Under Se adequate status, zebrafish skeletal muscle exhibits a low concentration of ROS, an active PI3K-Akt-TORC1 signalling and an efficient protein synthesis, which attribute to maintain the normal hypertrophy process of skeletal muscle fibres in zebrafish. (b) Under Se-deficient status, the down-regulation of antioxidant selenoproteins lead to a high concentration of ROS. The high concentration of ROS dephosphorylates and inactivate Akt, thereby inhibiting TORC1 pathway-mediated protein synthesis and leading to the suppressed hypertrophy of skeletal muscle fibres. IGF1, insulin-like growth factor 1; IRSs, insulin receptor substrates; PI3K, phosphatidylinositol-3-kinase; PIP2, phosphoinositide-4,5-biphosphate; PIP3, phosphoinositide-3,4,5-triphosphate; PDK1, phosphoinositide-dependent kinase 1; Akt, protein kinase B; TORC1, the target of rapamycin complex 1; TORC2, the target of rapamycin complex 2; ROS, reactive oxygen species.
Acknowledgements
This work was supported by the National Key Research and Development Program of China (X.Z., grant number 2019YFD0900303) and the Project funded by China Postdoctoral Science Foundation (L.W., grant number 2021M691171). These funders had no role in the design, analysis and writing of this article.
L. W. designed the study; J. Y., C. L., R. C., F. C. and H. Y. performed data acquisition and data analysis; L. W. and X. Z. wrote and reviewed the manuscript; X. Z. had primary responsibility for the final content. All authors have read and approved the final manuscript.
There are no conflicts of interest.